Inborn errors of metabolism
Crigler–Najjar syndrome type 1
Familial hypercholesterolaemia
FVII deficiency
Glycogen storage disease type I
Infantile Refsum’s disease
Progressive familial intra-hepatic cholestasis type 2 (PFIC2)
Urea cycle defects
Ornithine transcarbamylase deficiency
Arginosuccinate lyase deficiency
Carbamoyl phosphate synthase type 1 deficiency
Citrullinemia
Acute liver failure
Drug
Viral
Idiopathic
The aim of hepatocyte transplantation in metabolic disease is to replace the missing function without the need to replace the whole organ. In animal model of Crigler–Najjar syndrome, approximately 12 % of liver mass is needed to normalise the bilirubin levels [27]; however, fewer cells may be enough to change the phenotype in other conditions.
In acute liver failure, hepatocyte transplantation may act as a bridge to recovery and regeneration of the injured native liver or alternatively to orthotopic liver transplantation once an organ becomes available. The procedure may also be used in patients who are not candidates for organ transplantation. A major advantage of hepatocyte transplantation is the immediate availability of cryopreserved cells. Sufficient cell mass (approximately 10–15 % of liver cell mass) is needed to provide enough function to sustain metabolic function [27]. Hepatocyte transplantation in patients with ALF has resulted in a reduction in ammonia and bilirubin with improvements in hepatic encephalopathy and cardiovascular instability [19, 28, 29]. The advantages of intraperitoneal transplantation of hepatocytes encapsulated in alginate microbeads are currently being evaluated. Alginate serves as an anchorage point for cells and protects the hepatocytes from immune attack.
In metabolic liver diseases, the aim of hepatocyte transplantation is to replace function by the engrafted cells. Attempts at improving engraftment include temporary embolisation of the portal vein [30], liver irradiation [31] and the administration of growth factors [32].
The supply of good quality livers for hepatocyte isolation is a major issue. There is a pressing need for an easily available alternative cell source. Stem or progenitor cells have many advantages as an alternative source of cell for hepatocyte transplantation. They are readily available and may be effectively expanded in vitro or in vivo. Autologous cells (iPS cells) eliminate the need for immunosuppression; however, they may need to undergo corrective genetic manipulation prior to use to correct the underlying defect [33].
Early experimental evidence for progenitor cells/stem cells was demonstrated by Petersen [34], since that time there have been numerous studies showing expression of liver-specific genes by differentiated stem cells in vitro and in vivo summarised by a number of excellent reviews [33, 35, 36]. Proof of true functionality of these cells has been less frequent. It is not clear as yet whether transdifferentiation or fusion is responsible for the change in phenotype of the cells. For example, with severe selection pressure, such as in the fumarylacetoacetate hydrolase (FAH)– −/− –mouse (model for tyrosinemia type 1), successful repopulation occurs mainly because of hepatocyte fusion with host hepatocytes [37]. The tumorigenic potential of modifications to the genome needs to be considered.
Embryonic Stem Cells
Human embryonic stem cells (hESCs) , isolated in 1998 [38], have generated great interest and controversy over the past decade. Despite their undoubted therapeutic promise, their effective clinical application has remained elusive to date. hESC lines have been effectively differentiated to hepatocyte lineage with evidence of repopulation of immunosuppressed mouse model of liver injury [39, 40]. Undifferentiated ESC transplanted into immunodeficient mice resulted in teratoma formation [41, 42]. Safety concerns are thus a major obstacle to clinical translation. hESCs are thought to be less susceptible to immune rejection than more mature cells due to their low immunostimulatory potential [43], though the true capacity of hESC to evade the immune response remains unknown .
Hepatoblasts and Foetal Liver Progenitor Cells
The foetal liver is a source of more committed progenitor cells in the form of hepatoblasts. These cells retain their proliferative capacity but are ‘directed’ specifically towards development of hepatocytes and cholangiocytes. There has been success in using progenitor cells from foetal liver in animal models [44] and clinical case reports in ALF [45] and Crigler–Najjar syndrome [46]. Again there is some concern regarding the ability of progenitor cells to differentiate into functionally mature hepatocytes [47]. The most appropriate microenvironmental conditions for this have not yet been fully defined. An ‘adult’ microenvironment may be conducive to differentiation. Problems with this source include difficulty accessing tissue with uncertainty around the most appropriate gestational age to target. There are also some ethical concerns surrounding the harvesting of foetal tissue.
In contrast, ‘adult’ stem cells combine the ability to differentiate into liver cells with proliferative capacity. Use of this source is less ethically complex and closer to the clinic than embryonic or foetal liver stem cells.
Endogenous Liver Stem Cells
It is well known that the liver has the ability to regenerate by division of mature hepatocytes as demonstrated to good effect following partial hepatectomy [48] . Usually, hepatocytes turn over once or twice a year [36]. A second compartment of facultative stem cells become active in the presence of long-standing injury or impaired proliferation of mature hepatocytes. This occurs when replicative senescence is reached. These cells are located in the intrahepatic biliary tree within the canals of Herring [49], giving rise to bipotent cells which have the potential to become both cholangiocytes and hepatocytes. Adult-derived human liver stem cells have also been isolated by outgrowing cultures of primary hepatocytes and are thought to have advantages over other pluripotent cells. In particular, they are already ‘directed’ towards hepatocyte differentiation and thus are theoretically more likely to provide the required function without the problems of teratogenicity. A major advantage of these cells is their ability to expand in vitro. The functionality of these cells once differentiated has been documented [50].
Mesenchymal Stromal Cells
Mesenchymal stromal cells have also been shown to have promise in the cell therapy of liver disease [51]. These are multipotent, adherently growing cells which provide support for haematopoietic cells within the bone marrow [52]. They are a readily available source of stem cell also isolated from umbilical cord blood and matrix, placental tissue and adipose tissue [53–55]. Mesenchymal stem cells (MSCs) have beneficial effects in animal models of ALF. They can transdifferentiate into hepatocyte-like cells both in vitro and in vivo [56, 57]. MSCs play a major role in tissue repair both through localised immuneosuppressive effects and through the release of soluble trophic factors to affect neighbouring cells, properties which make them excellent candidates for improving the survival of transplanted cells [58, 59]. Improved survival has been shown in animal models of liver disease and pilot clinical studies of liver failure [60, 61]. It is not clear, however, whether the transdifferentiation of MSCs into hepatocyte-like cells or an anti-inflammatory, anti-apoptotic effect induced by the MSCs result in the desired outcome. Soluble factors such as growth factors, cytokines, extracellular matrix glycoproteins and other small molcules produced by MSCs likely mediate these effects in ALF rather than transdifferentiation [58]. Candidate molecules include interleukin (IL)-1Rα, IL6, IL8, granulocyte-colony-stimulating factor, nerve growth factor and hepatocyte growth factor [60, 62]. MSC-conditioned medium conferred a significant improvement in mortality and biochemical indices of liver damage in a galactosamine-injured rat with evidence of inhibition of apoptosis and promotion of liver regeneration in the treated liver [63]. This anti-apoptotic, pro-regenerative effect of MSCs has also been seen in the setting of myocardial infarction [64] and stroke [65]. In addition to the beneficial effects on liver inflammation and regeneration, other studies have recognised the supportive effects of bone-marrow-derived cells in coculture with hepatocytes [66]. Co-encapsulated hepatocytes and bone marrow stem cells resulted in prolonged maintenance of function in the co-encapsulated group in vitro and in vivo in a rodent model of ALF [67, 68]. Thus, not only are MSCs anticipated to improve the viability and functionality of co-transplanted hepatocytes but transplanted mesenchymal stem cells may also be a source of anti-inflammatory/anti-apoptotic mediators in the setting of the failing liver and the subsequent cytokine storm. MSCs are already used clinically in the treatment of graft versus host disease, and these have safety and logistical advantages over hESC and alcoholic liver disease/hepatic stellate cell ALD/HSC.
Induced Pluripotent Stem Cells
The description by Takahashi and Yamanaka [69, 70] of the induction of somatic cells back to their pluripotent state by over-expressing certain transcription factors, and the subsequent differentiation of the cells along alternative lineages, has opened massive possibilities for cell therapy in liver disease . The differentiation of autologous dermal cells into hepatocytes following induction of pluripotency may have significant advantages, avoiding the need for immunosuppression in cell transplantation and providing an accurate model of disease to investigate pathophysiological mechanisms and test therapies. Of course, the transplantation of autologous cells in a disease state would require the need for genetic manipulation to correct that defect in the first instance. Thus, potential genomic instability may be a problem; however, new techniques are emerging which may minimise this risk and allow genomic stability. One such approach is using a piggyBac technique which enables removal of transgenes without residual sequences. This technique was used to good effect in a study by Yusa et al. which differentiated iPS cells from dermal cells of a patient with alpha-1-antitrypsin deficiency, corrected the genetic abnormality and demonstrated the function of the hepatocyte-like cells when transplanted into a mouse [15]. iPS-derived hepatocyte cells have also been used in the successful formation of ‘liver buds’ together with endothelial cells from umbilical cord and mesenchymal stromal cells. When transplanted into a mouse, this human liver bud rapidly vascularised and demonstrated liver-specific function [71] .
Immortalisation
In order to establish a sustainable source of cells for transplantation, immortalisation of hepatocytes has also been described. Clonal cell lines of human and rat hepatocytes have been transduced with retroviral vector expressing the immortalising simian virus 40 large T antigen gene [72]. Despite control mechanisms for switching immortalisation on and off, concerns regarding safety remain.
Thus, the potential of cell therapy for liver disease is promising, though cell source is an issue, the emergence of mesenchymal stromal cells and iPS cells into the clinical field is particularly relevant and may provide an alternative source for successful translation. Pediatric liver disease, especially liver-based metabolic disease, remains a priority for this approach.
Small Molecules and Chaperones
As a reduction in protein stability is often a common outcome of monogenic disease, for example, alpha-1-antitrypsin deficiency or cystic fibrosis , focus on the stabilisation of such proteins and provision of an increase in intracellular function is the remit of pharmacological chaperones and other small molecules .
The work of Lomas group and others have focused on the use of small molecules to block the abnormal polymerisation of alpha-1-antitrypsin in the Z state [73]. At present, however, this work is proof of principle and not yet at translation point, though in the future may offer real therapeutic promise. A pediatric group in Pittsburgh investigated the use of carbamazepine as an autophagy enhancer to clear the intracellular load of abnormally formed alpha-1-antitrypsin in the disease state and reduced liver fibrosis in mice [74], using a well-established pharmacological substance as a molecular manipulator. Similarly, lysosomal storage disease and phenylketonuria have also been a focus for the development of protein chaperones [75, 76] .
Targeting other nuclear receptors with pharmacological agents is a focus for polygenic or multifactorial disease such as NAFLD and primary biliary cirrhosis. Farnesoid X receptor (FXR) is a nuclear receptor that binds extensively to genomic DNA. FXR undergoes conformational change and alters gene expression following binding by bile acids or other pharmacological compounds. This results in the regulation of bile acid levels in the liver and in addition glucose and lipid homeostasis. Thus, modulation of FXR binding and function using a semi-synthetic bile acid may be a useful approach to therapy of nonalcoholic steatohepatitis (NASH) [77] and certain cholestatic liver diseases for which there are early clinical studies ongoing at present [78].
Conclusions
Pediatric hepatology is increasingly becoming an independent speciality. Success of liver transplantation has been crucial to the success of this speciality. A majority of chronic liver diseases are of perinatal onset with large number due to genetic or metabolic defects. Rapid advances in the field of genetics and molecular medicine will help unravel the mechanisms underlying these disorders and molecular diagnostic approaches. Understanding the underlying mechanism will help us to use newer treatments like gene therapy and small molecules . In parallel, the field of cellular therapy using human hepatocytes continues to gain momentum with proof-of-concept clinical applications in liver-based metabolic disorders and ALF. Stem cell sources of liver cells from embryonic stem cells, induced pluripotent cells and production of liver organoids from stem cells with use of growth factors appear to hold great promise to replace whole liver replacement in future.
References
1.
2.
Starzl TE, Marchioro TL, Vonkaulla KN, Hermann G, Brittain RS, Waddell WR. Homotransplantation of the liver in humans. Surg Gynecol Obstetr. 1963;117:659–76 (PubMed PMID: 14100514. Pubmed Central PMCID: 2634660).
3.
4.
5.
6.
7.
Sambrotta M, Strautnieks S, Papouli E, Rushton P, Clark BE, Parry DA, et al. Mutations in TJP2 cause progressive cholestatic liver disease. Nat Genet. 2014;46(4):326–8 (PubMed PMID: 24614073).PubMedCentralCrossRefPubMed
8.
de Vree JM, Jacquemin E, Sturm E, Cresteil D, Bosma PJ, Aten J, et al. Mutations in the MDR3 gene cause progressive familial intrahepatic cholestasis. Proc Natl Acad Sci U S A. 1998;95(1):282–7 (PubMed PMID: 9419367. Pubmed Central PMCID: 18201).PubMedCentralCrossRefPubMed
9.
Strautnieks SS, Kagalwalla AF, Tanner MS, Knisely AS, Bull L, Freimer N, et al. Identification of a locus for progressive familial intrahepatic cholestasis PFIC2 on chromosome 2q24. Am J Hum Genet. 1997;61(3):630–3 (PubMed PMID: 9326328. Pubmed Central PMCID: 1715942).PubMedCentralCrossRefPubMed
< div class='tao-gold-member'>
Only gold members can continue reading. Log In or Register a > to continue
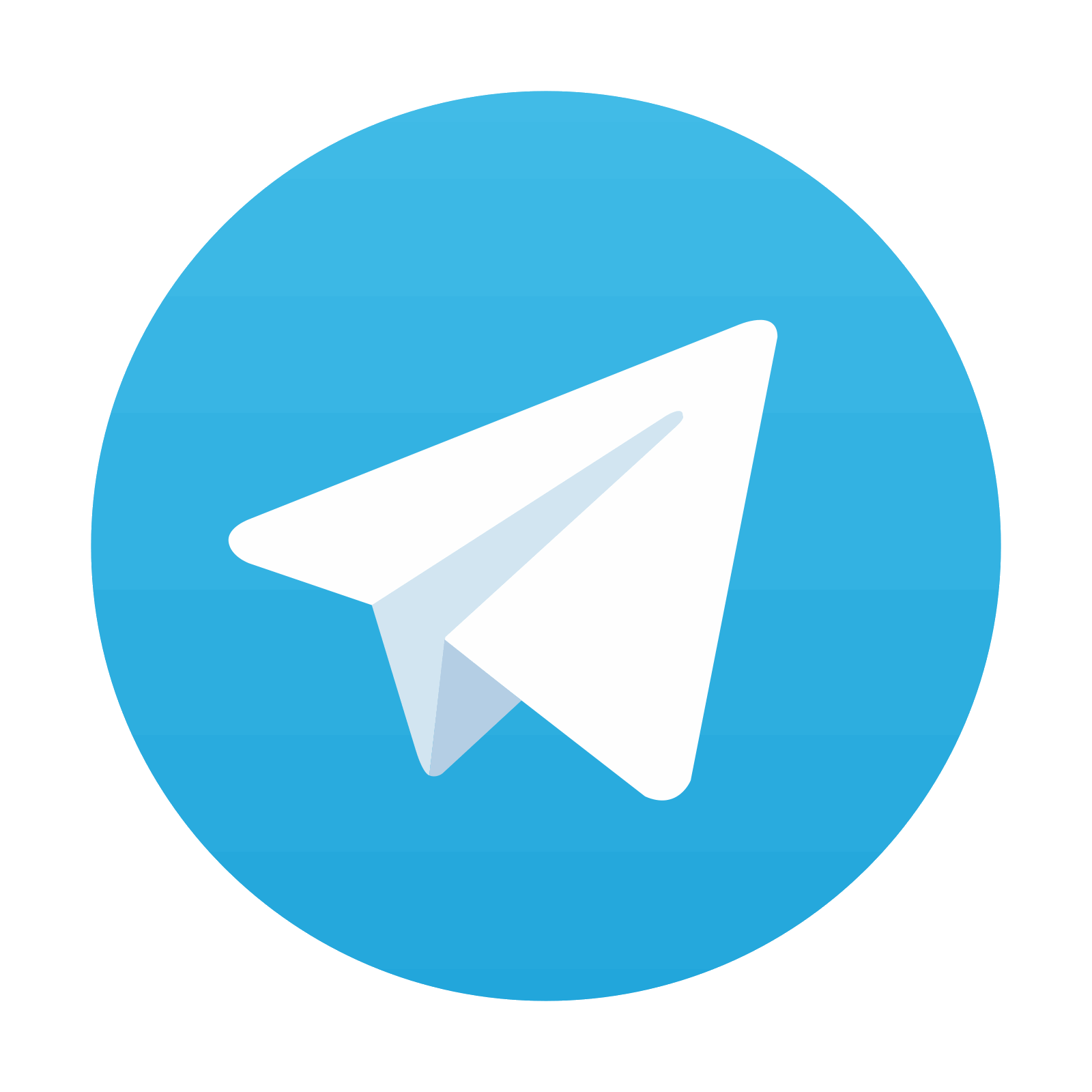
Stay updated, free articles. Join our Telegram channel
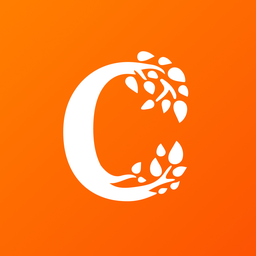
Full access? Get Clinical Tree
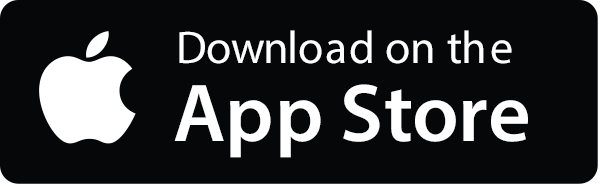
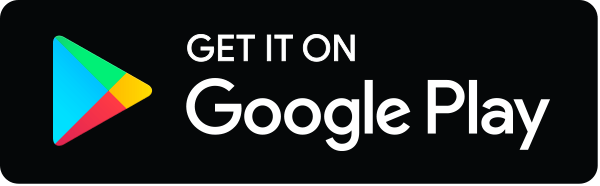