Fig. 21.1
Hypothetical scheme of ammonia-HE-induced astrocyte swelling. Note the interconversion between ammonia and glutamine and that both result in the formation of ROS. While the role of aquaporin-4 (AQP-4) in this scheme is uncertain, inhibition of the MPT was able to block the upregulation of AQP-4 by ammonia. (GS glutamine synthetase, MPT mitochondrial permeability transition, PAG phosphate-activated glutaminase, PBR peripheral benzodiazepine receptor, ROS reactive oxygen species.)
Through undefined mechanisms, neurotoxins, especially ammonia, induce changes in neurotransmission. The peripheral-type benzodiazepine receptor (PTBR) in particular is thought to contribute to HE pathogenesis [6]. Increased PTBR signaling leads to increased cholesterol uptake and neurosteroid synthesis. Neurosteroids such as allopregnanolone act as positive allosteric modulators of the GABAA receptor [7]. The net result is a significant increase in GABA-ergic tone [8].
In addition to altered inhibitory neurotransmission, alterations in excitatory neurotransmission are seen in experimental models and patients with HE as well. Interestingly, despite a total decrease in total brain glutamate, extracellular glutamate is increased in animal models of HE [9–11]. The increase in extracellular glutamate is thought to be caused by astrocytic dysfunction leading to decreased glutamate reuptake or excessive neuronal release of glutamate due to depolarization via ammonia [12, 13]. Increased NMDA signaling has also been implicated in the pathogenesis of HE by increasing sodium influx and subsequent cellular swelling [14].
Systemic inflammation and neuroinflammation also play critical roles in the pathogenesis of HE [15]. The development of systemic inflammatory response syndrome (SIRS) is a potent predictor of developing HE. Circulating tumor necrosis factor alpha (TNF-α) levels are also correlated with the severity of HE and known polymorphisms of TNF are known to influence prognosis [16, 17]. Studies have also shown that microglia are activated in HE, providing direct evidence of neuroinflammation [18]. Once activated, microglia produce inflammatory cytokines, especially TNF-α, interleukin (IL)-1β, and IL-6. Deletion of either the gene encoding TNF or IL-1β resulted in a delay in the onset of HE and attenuated cerebral edema [19]. There remains debate over how the integrity of the BBB changes in response to this proinflammatory setting and the associated effects on HE and cerebral edema [20].
Intracranial Hypertension
HE associated with acute liver failure and cirrhosis can result in intracranial hypertension. Intracranial hypertension is defined as intracranial pressure (ICP) exceeding 20 mmHg. ICP is determined by the brain, blood, and cerebrospinal fluid (CSF). Brain parenchyma composes 80% of the contents of the skull while blood and cerebrospinal fluid account for approximately 10% each [21]. ICP increases with an increase in the volume of the brain parenchyma, which can occur with an expanding mass or an increase in cerebral water content, an increase in blood volume, which may occur when cerebral autoregulation is impaired, or an increase in CSF volume either due to increased production or impaired resorption [22]. The driving force for blood flow within the brain is the cerebral perfusion pressure (CPP). Cerebral perfusion pressure (CPP) is dependent upon the mean arterial pressure (MAP) and ICP. More specifically, CPP is equal to the ICP subtracted from the MAP (i.e., CPP = MAP – ICP). Thus if the MAP is held constant, as ICP increases, cerebral perfusion pressure (and consequently blood flow) decreases, increasing the risk for ischemia. ICP elevation can also result in various herniation syndromes that are frequently fatal.
In HE, ammonia is converted to glutamine within astrocytes, as discussed previously. As glutamine is osmotically active, this results in astrocytic swelling and is a primary contributor to cerebral edema in HE [23]. Proinflammatory signaling molecules may also contribute to astrocyte swelling and increased ICP [24]. A number of other factors are thought to mediate the effects of ammonia and inflammatory signals, including reactive oxygen species (ROS), nuclear factor kappa B (NF-κB) signaling, mitogen activated protein kinase (MAPK) signaling, mitochondrial permeability transition, and various ion transporters and aquaporins [25, 26]. Consequently, great care must be taken to manage cerebral perfusion pressure, cerebral blood volume, and cerebral blood flow to reduce the risk of herniation or ischemia [27].
Clinical Manifestations
Hepatic encephalopathy presents clinically as a wide spectrum of cognitive and neuromuscular dysfunction. HE is classified by the underlying etiology, clinical signs and symptoms, the time course, and precipitating factors [28]. Type A HE occurs in the setting of acute liver failure, type B HE occurs in the setting of porto-systemic bypass with no intrinsic hepatic disease, and type C HE occurs in the setting of cirrhosis. The time course is classified as episodic, recurrent, or persistent. In patients with acute liver failure (i.e., type A HE), the onset of symptoms is typically sudden and patients rapidly progress through the grades of HE. Type A HE is associated with elevated ICP and frequently results in death within hours to days of symptom onset [29]. HE in the setting of chronic liver disease (i.e., type C HE) is typically more insidious and often occurs episodically.
The most common grading system to classify the clinical manifestations of HE is the West Haven Criteria (WHC) [28]. Patients with minimal hepatic encephalopathy (MHE), sometimes referred to as Grade 0 HE, demonstrate limited clinically recognizable signs and may often only be identified following abnormal neuropsychological testing [30]. Sleep disturbances, both insomnia and hypersomnia, are frequently an initial manifestation of HE [31]. Furthermore, the absence of excessive daytime sleepiness has a strong negative predictive value for the development of HE in patients with cirrhosis [32].
Patients with grade I HE typically present with impaired attention and increased difficulty with basic arithmetic and other similar cognitive skills. Neuromuscular findings in these patients include mild tremor, slurred speech, and impaired handwriting [33]. Asterixis may also become evident in patients with grade I HE. Grade I HE is associated with behavioral changes including irritability, depression, or euphoria [34].
Grade II HE results in further cognitive impairment, including amnesia and gross deficits in basic computation. Behaviorally, patients may exhibit disorientation or inappropriate behavior in addition to marked lethargy. Grade II HE is also characterized by more prominent asterixis, hypoactive reflexes, and muscular rigidity [35].
Patients with grade III HE demonstrate somnolence and significant confusion, typically disorientation to time and place. Neuromuscular dysfunction is especially pronounced and may result in incontinence, incoherent speech, hyperreflexia, clonus, and a positive Babinski’s sign [34].
In grade IV HE, patients are comatose and may or may not respond to noxious stimuli. Decerebrate posturing is also seen in these patients. Although infrequent, focal neurologic deficits may also be seen in patients with HE, most commonly hemiplegia [36].
Diagnosis
The diagnosis of HE starts with a thorough history and physical examination to identify (1) the characteristic signs and symptoms of HE, (2) the evaluation of other possible causes of altered mental status, (3) the evaluation of possible precipitating factors, and (4) response to empiric therapy. Several diagnostic tools have been developed to aid in the process of identifying patients with HE. Among these tools are the hepatic encephalopathy scoring algorithm (HESA), clinical hepatic encephalopathy staging scale (CHESS), and modified-orientation log (MO-log) [37–39]. While these various tools provide an objective measure of severity and can increase the precision with which HE is diagnosed and classified, which is particularly useful in therapeutic trials, their clinical use is often limited due to concerns about the length of time it takes to administer these tests.
There is limited utility in additional laboratory testing or imaging in the evaluation of HE. The levels of ammonia, which play in important role in HE pathogenesis, are consistently elevated in patients with HE [40]. The correlation between the degree of hepatic encephalopathy and arterial ammonia levels only holds true for ammonia concentrations up to two times the upper limit of normal [41]. A potential alternative laboratory biomarker for MHE is 3-nitrotyrosine, a product of protein nitrosylation via peroxynitrite, a derivative of nitric oxide, which is elevated in MHE. 3-Nitrotyrosine was shown to have a sensitivity of 93% and a specificity of 89% in the diagnosis of MHE in patients with cirrhosis [5, 42].
Pharmacologic Treatment-Encephalopathy
The treatment of overt HE consists of managing precipitating factors, ammonia reducing therapies, reduction of elevated ICP, and prevention of recurrent bouts of HE. Correction or treatment of the precipitating cause of HE is typically associated with improvement or resolution of HE [43]. Common precipitating causes that should be investigated and treated include gastrointestinal (GI) bleeding, infection, metabolic alkalosis, hypovolemia, hypoglycemia, and renal failure. The mainstay of HE therapy is the reduction of ammonia. Lactulose and lactitol, both nonabsorbable disaccharides, are first-line therapies [44].
Antibiotics also play an important role in the treatment of overt HE. Antibiotics improve HE through the elimination of ammonia producing bacteria that reside in the GI tract. The current antibiotic of choice is rifaximin [45]. In patients with overt HE, rifaximin has been shown to be more efficacious than lactulose therapy in both treating HE and improving patient reported quality of life [46]. Furthermore, randomized, placebo-controlled studies of rifaximin in patients already receiving lactulose showed that rifaximin therapy was more effective than placebo as a secondary prevention measure to reduce recurrent bouts of HE [47].
Nutritional support is also essential in the management of HE. In the past, patients with HE were placed on protein restricted diets under the assumption that it would reduce the total amount of ammonia absorbed through the gut and improve HE [48]. However, in patients with chronic liver disease and HE, protein restriction exacerbates malnutrition and increases mortality compared to patients with a diet containing protein [49]. Current recommendations are for a diet that provides 35–40 kcal/kg/day, including 1.2–1.5 g/kg/day of protein [50]. In addition, corrections of zinc deficiency and supplementation with l-ornithine-l-aspartate, both necessary components of the urea cycle, have shown benefits in treating HE and reducing circulating ammonia [51, 52]. Branched-chain amino acids have also shown a therapeutic benefit in HE, but the data do not show any survival advantage [53, 54].
Feasibility of Invasive Intracranial Monitoring
An estimated 86–95% of patients with grade III–IV encephalopathy have intracranial hypertension [61]. Moreover, complications from intracranial hypertension account for a large portion of death in patients with acute liver failure, as an estimate is likely around 20–35% [62]. There is some debate in terms of whether implanting an invasive intracranial pressure (ICP) device is necessary, where some centers suggest that patients are managed optimally without it while others find that invasive ICP monitoring can be beneficial [63, 64].
The frequency of invasive ICP monitoring varies widely among different institutions. For example, Vaquero et al. found that 28% of the studied cohort among 24 different centers used ICP monitoring [65]. However, the variability is high among different centers globally, possibly due to the perceived high potential of serious complications [66]. Indeed, frequency of ICP monitoring can range from 0 to 85% in the USA, depending on institution [65].
Screening patients for the risk of intracranial hypertension is complex and involves a number of factors. Those with cerebral edema will likely also suffer from intracranial hypertension. Risk factors for cerebral edema include (1) a high-grade hepatic encephalopathy (e.g., grade III or IV), (2) elevated serum ammonia levels (i.e., higher than 100 μmol/L), (3) a rapidly progressive deterioration of hepatic function (i.e., progression from jaundice to encephalopathy in less than 1 week), (4) the presence of a systemic inflammatory response syndrome (SIRS), and (5) a requirement for vasopressors or renal replacement therapy [67, 68]. Intracranial hypertension should be suspected in AFL patients who experience newly onset hypertension, worsening encephalopathy, or deterioration in their neurological examination [62]. Furthermore, risk of edema increases to 25–35% with progression to grade III and 65–75% or higher in patients reaching grade IV encephalopathy [69].
Imaging is usually inadequate in diagnosing intracranial hypertension or cerebral edema. For example, computed tomography (CT) imaging of the brain is usually not sensitive enough to determine intracranial hypertension or rule out cerebral edema [62]. There are specific markers in CT findings that are suggestive of cerebral edema (e.g., sulci effacement, compression of basal cisterns, midline shifts), but the absence of these does not exclude the possibility of cerebral edema [70, 71]. Rather, CT imaging in ALF patients is used to rule out pathology other than intracranial hypertension or cerebral edema (e.g., intracranial hemorrhage, hydrocephalus) [72]. Magnetic resonance imaging (MRI) has not been shown to properly assess cerebral edema in ALF patients most likely because of the prolonged time required for obtaining images [62].
Advantages of Invasive Intracranial Monitoring
The benefits of invasive ICP monitoring are the following: (1) ICP can be monitored accurately and precisely, allowing for quick detection of elevated ICP, (2) it can allow for an immediate evaluation of ICP response to therapy, (3) it provides knowledge of intracranial perfusion pressure, (4) it provides information that allows for accurate patient prognosis, and (5) anesthetic management during liver transplant is improved with ICP monitoring because ICP surges intraoperatively are common [73]. Ultimately, these benefits may allow for longer patient survival prior to transplant, which would increase the chance allocating a graft [73].
The main goal of monitoring ICP is to maintain appropriate cerebral profusion. Ideally, this means keeping ICP below 20 mmHg and CPP above 70 mmHg [62]. When patients are monitored, they may be more likely to receive appropriate treatment in a timely manner (e.g., mannitol, barbituates, vasopressors) [65]. Recommendations of the US Acute Liver Failure Study Group for the intensive care of patients with ALF state that ICP monitoring should be considered in all patients listed for liver transplantation with grade III/IV hepatic encephalopathy, and in patients with advanced hepatic encephalopathy who are not transplant candidates but have reasonable chances of recovery (e.g., patients with acetaminophen toxicity ALF) [74].
While there are no published reports listing cut-off criteria with regards to ICP and liver transplant Vaquero et al. found that patients who have invasive monitors are more likely to receive vasopressors and ICP-related medications [65]. However, they also found no difference in 30-day survival between liver transplant patients who undergo invasive monitoring and those that do not. They theorize that the real benefit of ICP monitoring is not in the 30-day survival but possibly in the impact on long-term neurological recovery following liver transplant [65].
Safety of Intracranial Monitoring
The main risk associated with invasive ICP monitoring is bleeding because of the coagulopathy associated with liver failure. Arguably, intracranial hemorrhage though is the main complication that prevents invasive ICP monitoring from being used widely in the care of ALF patients [62]. A study showed that bleeding occurred in 20% of patients, where 5% of patients had a fatal hemorrhage; moreover, invasively monitored patients did not experience improved outcomes when compared to the control [75]. However, these percentages are inconsistent. Another study showed that out of 92 patients who were invasively monitored, the rate of hemorrhages for roughly 10% (half of them found incidentally via imaging) with only two deaths [65]. Further, another study of 101 patients showed there was no significant difference in mortality between ALF patients who were invasively monitored and those who were not [76]. Although hemorrhage is a serious risk of invasive ICP monitoring, its likelihood is probably determined by protocol and management.
Why Is Invasive Intracranial Monitoring Not Associated with Better Outcomes?
There is little evidence associating invasive ICP monitoring with better outcomes. There could be a number of hypotheses to explain why, as described by Bernuau and Durand [66]. First, the risks associated with implanting the device may offset the potential benefits that are conferred by ICP monitoring. Second, there is no established standardized algorithm for determining which patients require invasive ICP monitoring, thus there likely has not been accurate investigation of the cohort whom ICP monitoring could actually help. Third, invasive monitoring itself is not therapeutic and thus not directly beneficial, which means that the benefits exacted are likely to be more mild and modest in comparison to a direct therapy.
As previously mentioned, supportive evidence for the use of invasive ICP monitoring may be lacking because patient selection is likely crucial. For example, a retrospective study investigating ICP monitoring in ALF patients with high-grade encephalopathy found that in patients with ALF due to acetaminophen toxicity, ICP monitoring did not impact 21-day mortality, while ICP monitoring was associated with high 21-day mortality in patients with ALF not associated with acetaminophen toxicity [77]. Conversely, another study showed that ICP monitoring resulted in higher survival rates in pediatric patients in comparison to those who were not monitored while maintaining low complication rates [78]. Therefore, ICP monitoring is likely beneficial in a specific patient population while detrimental in another and the specific characteristics of either has yet to be fully elucidated.
Indications for Invasive ICP Monitoring
There are a number of factors that are incorporated into the algorithm for deciding who should undergo invasive ICP monitoring. Gasco et al. state that based on their review of the literature, invasive monitoring is based on level 2 evidence, at best [79]. Generally, patients who have liver failure and known elevated ICP, as graded by an ammonia levels >200 μmol/L, grade III or IV encephalopathy, acute renal failure, or a need for vasopressor support [80]. CT scans likely do not provide reliable evidence that demonstrates cerebral edema, especially during the early grades [70]. Further, the American Association for the Study of Liver Diseases established a recommendation that invasive ICP monitoring should be considered in patients with high-grade hepatic encephalopathy (e.g., grade III or IV) if their care center has experience with ICP monitoring or patients who are awaiting or undergoing liver transplantation [81]. After transplantation, intracranial hypertension could be more likely to develop because rapid shifts in electrolytes and hemodynamic changes associated with surgery may cause large ICP fluctuations [80].
Types of Invasive Intracranial Monitoring
Different methods are typically dependent on the anatomic location of the reading, which include intraventricular, intraparenchymal, epidural, subdural, and subarachnoid. External ventricular drainage (EVD), where a catheter is placed into a ventricle, is considered to be the most accurate method [82]. EVD placement is not considered to be a major procedure, but it does carry the highest risk of hemorrhage or infection [83]. Although some believe EVD to be the most beneficial monitor because they allow the potential therapeutic option of CSF drainage [85]. ICP microtransducers are widely used and can be divided into multiple categories (e.g., fiber optic devices, strain gauge, pneumatic sensors) [83]. Using microtransducers to measure intraparenchymally or intraventricularly is most common, as epidural and subdural measurements are typically not as accurate. For example, a study showed that epidural measurements consistently overestimated ICP [84]. However, intraparenchymal or intraventricular measurements are not always possible, thus the most accurate method that does not jeopardize patient safety should be utilized. A sterilized environment should always be used to minimize the risk of infection. Prophylactic antibiotics may be considered, though there is the concern of infection from drug-resistant organisms.
Liver Failure ICP Monitoring Protocol
In collaboration with the Department of Neurosurgery, the Cleveland Clinic has developed the following algorithm for invasive ICP monitoring of liver failure patients (Fig. 21.2). Patients must be under consideration for liver transplantation and be in grade III or IV coma secondary to liver failure. First, invasive procedures (e.g., central lines, implantation of an ICP monitoring device) should be scheduled and performed as close together temporally as possible. Second, critical care and hepatology should do a preliminary discussion with the patient’s family regarding the rationale behind implanting the ICP monitoring device, but formal consent should be obtained by neurosurgery. Critical care must be consulted to determine the optimal efficacy of implantation and subsequent management of the ICP monitor. Third, the implantation should be done at the bedside regardless of INR. Fourth, if the platelet count is under 80,000 platelets/μL, platelets should be administered. Fifth, one unit of FFP should be administered for at least 10 min in preparation for the procedure and another unit should be administered for 20 min simultaneously with the procedure. One unit of FPP should be administered at least 20 min after the procedure. Sixth, if a hematoma develops after implantation of the monitor, surgical evacuation should not be offered if the INR is greater than 1.3 or the platelet count is fewer than 100,000 platelets/μL.


Fig. 21.2
Cleveland Clinic Algorithm for ICP monitor insertion in liver failure
The use of FFP may need to be adjusted depending on the patient. Study of the USALF showed that FFP was most common in 91% of patients and FPP was used in conjunction with another treatment in 59% of patients; others (e.g., platelets, cryoprecipitate, and factor VIIa) were used 18–31% of the time [65].
Traditionally, coagulopathy was managed by infusion of FFP, platelets, and vitamin K, but more recent evidence has suggested that administering recombinant factor VIIa may help optimally minimize the risk of bleeding [86, 87]. Le et al. reported a case series of 11 patients who received recombinant factor VIIa prior to ICP monitor implantation and they reported that there were no complications associated with invasive monitoring [87]. However, they had a small sample size and did not repeat laboratory findings after factor VIIa was administered [87]. It is possible that because factor VIIa has a relatively short half-life (2–5 h), it is the first factor to become deficient in liver failure patients, and thus replenishing it likely helps to play a role in preventing bleeding complications [86]. Another study compared eight liver failure patients who received only FFP and seven who received FFP and recombinant factor VIIa and found that only 38% of the patients who received FFP had acceptable laboratory values that allowed for ICP monitoring while all of the patients who received FFP and recombinant factor VIIa had acceptable laboratory values for ICP monitoring [88]. The study also found that those who received the recombinant factor VIIa were also less likely to develop anasarca in comparison with those who did not; the authors hypothesized that this was due to better fluid management secondary to data provided by ICP monitoring and less high volume FFP required to reverse coagulopathy [88].
Managing Invasive ICP Monitoring
The cerebral perfusion pressure (CPP) is the intracranial pressure (ICP) subtracted from the mean arterial pressure (MAP) (i.e., CPP = MAP – ICP); therefore to maintain adequate CPP, clinicians can either increase MAP or decrease ICP. Practically, CPP can be maintained by (1) optimizing the patient’s position and (2) appropriate pharmacologic management. Optimizing the patient’s position means that the head of the bed should be elevated over 30° (i.e., putting the bed in a sitting position) with nothing compressing the neck and protecting the blood flow through the jugular veins. Hyperventilation for short periods can also help by causing vasoconstriction.
In terms of pharmacologic agents, indomethacin can cause vasoconstriction that may reduce ICP while maintaining adequate perfusion and causing no significant changes in cerebral microdialysis [89]. There are potential risks though, such as nephrotoxicity, platelet dysfunction, or GI bleeding/ischemia [89]. Additionally, osmotic diuretics can help decrease ICP pressure by increasing blood osmolality, which draws fluid out of the cerebral tissue and into the intravascular space (i.e., decreasing volume of fluid in the brain). For example, administrating mannitol has been shown to reduce ICP and increase survival among grade IV encephalopathic ALF patients [90]. Further, hypertonic saline solutions and propofol may also help lower ICP in patients with IV encephalopathic ALF patients [91, 92]. Pentobarbital is typically not used in ALF patients due to prolonged clearance [62].
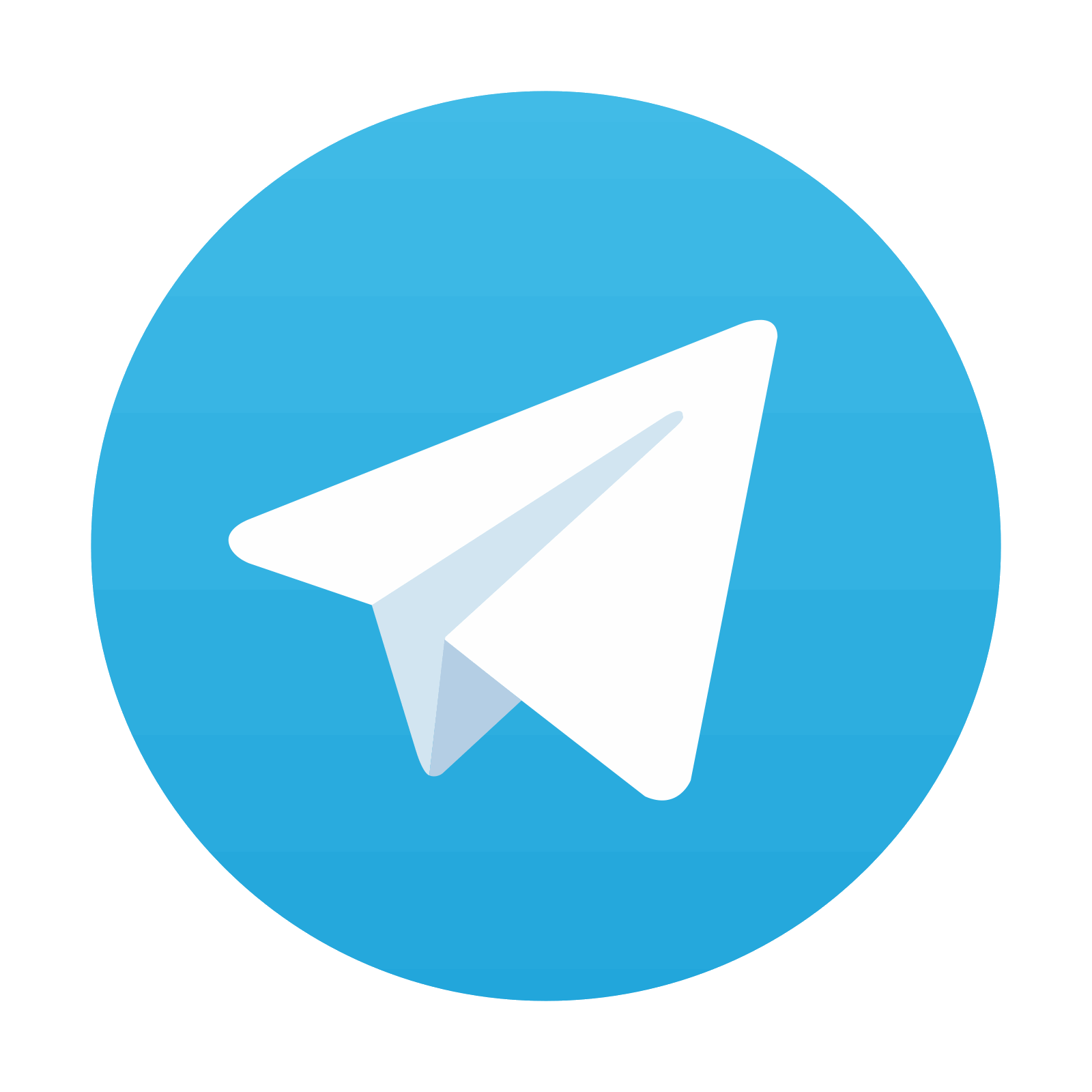
Stay updated, free articles. Join our Telegram channel
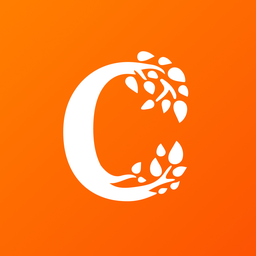
Full access? Get Clinical Tree
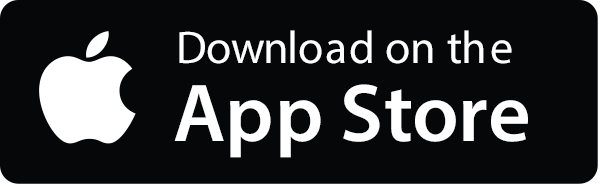
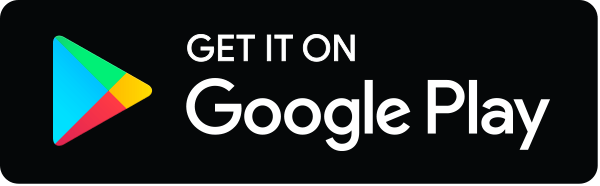