Abstract
The transport functions of epithelial cells in the gastrointestinal (GI) tract, and elsewhere, are made possible by their remarkable apical-basolateral plasma membrane asymmetry. This property, commonly called membrane polarity, is achieved by a combination of intracellular trafficking operations, including sorting, vectorial delivery and membrane-specific docking, fusion and retention mechanisms. Here we provide a review of this rapidly evolving research field within a historically relevant context.
Keywords
Epithelial polarity, Protein sorting, Sorting signals, Adaptins, SNARES, Rab GTPases
45.1
Introduction to Epithelial Cell Polarity
The ability of epithelial cells in gastrointestinal (GI) track to absorb selected nutrients while secreting electrolytes, enzymes, and digestive factors depends on intracellular trafficking mechanisms that establish and maintain the polarized disruption of different transport proteins and surface receptors on the apical and basolateral membranes. A combination of intracellular sorting operations, vectorial delivery mechanisms, plasmalemma-specific fusion, and retention processes are responsible. Well-defined signals that specify polarized sorting or retention of proteins have been described, and the intracellular machinery that decode and act on these signals are becoming better understood. In this chapter, we provide an up-to-date review of the trafficking routes, molecular machinery, and mechanisms involved in protein sorting and trafficking.
45.2
Cytoarchitecture and Membrane Compartments in Polarized Epithelial Cells
Epithelial cells exhibit tremendous diversity and specialization along the GI tract, yet share many common morphological features. These include a basement membrane, a complex set of cell junctions that demarcate the boundary between the apical and the basolateral membrane, a cytoskeleton with a specific spatial distribution, and a set of membrane compartments that serve as platforms for polarized sorting and delivery of the apical and basolateral membrane proteins. Although a detailed description of these structures exceeds the purpose of this chapter, a brief summary of their distribution and functional significance is highlighted to provide a context for understanding the mechanisms of membrane polarity (see Fig. 45.1 ).

45.2.1
Epithelial Cell Junctions
The tight junction complex at the apex of the cell forms a relatively impermeable barrier between the solutions on either side of the epithelium, and acts as a molecular “fence” to partition the contents of the apical and basolateral membranes from each other. . It is formed of integral membrane proteins, such as occludin, the claudins, and the junction adhesion molecule (JAM), which assemble as a network of strands and interact with opposing membranes of adjacent cells through their extracellular domains. A number of regulatory and scaffolding proteins associate with the cytoplasmic surface of the tight junction, including ZO-1, -2, and -3, cingulin, 7H6 and symplekin, providing a means to connect the junction to the actin cytoskeleton. They also allow recruitment of signal transduction proteins and polarity generating complexes, creating de facto signaling platforms. Importantly, components of the exocyst, a multiprotein complex involved in polarized protein trafficking, also associate with or near tight junctions.
The adhesion between epithelial cells is mostly due to the Zonula adherens , a belt-like structure that encircles the cell just below the tight junctions. Cadherins are the main component of the Z. adherens , with their extracellular domains likely mediating a calcium-dependent trans-dimerization process that provides adhesion between neighboring epithelial cells. In all, the apical junctional complexes are dynamic structures that undergo dramatic rearrangement and redistribution during embryonic development, cell migration, and proliferation, closely integrating these processes to an equally dynamic cytoskeleton. The exocyst plays an important role in maintaining adherens junctions. In intestinal epithelia, cholera toxin inhibits exocyst-mediated trafficking of E-cadherin, thus compromising epithelium barrier integrity, which contributes to diarrhea. In addition to the apical junctional complexes, desmosomes, composed of desmosomal cadherins, associate to intermediate filament (IF) attachment proteins and provide additional anchoring points throughout the basolateral membranes, contributing to the maintenance of the epithelium integrity.
45.2.2
The Cytoskeleton in Epithelial Cells
The cytoskeleton in epithelial cells is closely associated with both the apical and basolateral membranes, playing important structural and functional roles in protein sorting and trafficking. The building blocks of the cytoskeleton, namely microfilaments (MF), microtubules (MT), and IF, are organized as depicted in Fig. 45.1 (reviewed in Ref. ). The MF are formed by the polymerization of β- and γ-actin monomers, with a fast-growing plus-end (“barbed”) and slow-growing minus end (“pointed”). Multiple MF associate to form filamentous networks, for which some cross-linking proteins such as α-actinin or filamin are required. In addition, other actin-binding proteins control polymerization dynamics, bundling, nucleation, branching, actin-membrane interaction, cell-extracellular matrix (ECM) interaction, contractility, scaffolding, and signaling. MT are composed of α- and β-tubulin monomers that combine to produce αβ-dimers, prior to being added in a head-to-tail fashion to the fast growing plus-end of an existing MT. This intrinsic polarity is of great importance in defining the directionality of their transport capabilities. In epithelial cells (such as Caco-2, MDCK, or enterocytes) MT emanate from multiple noncentrosomal organizing centers (MTOC) located below the apical membrane (pericanalicular region in hepatocytes), and assemble into polarized bundles running along the apical-to-basolateral axis, with their rapidly changing plus-ends projecting toward the basal membrane. However, a group of MT is oriented with their plus-ends toward the apical membrane and appears to play an important role in polarized vesicle transport in MDCK cells (see Section 45.7.1 ). IF in intestinal epithelial cells are composed mostly of keratin types 8, 18, and 19, and have important structural roles.
At the apex of the lateral membranes, running along the cell-adhesion junction, densely packed antiparallel arrays of actin filaments associate to myosin II in order to generate a contractile ring. This structure is closely associated with the apical junctional complexes via E-cadherin interactions, and creates an extensive transcellular network important, for example, in canalicular contraction during bile secretion. Underling the apical membrane, a meshwork of parallel arrays of actin filaments cross-linked by myosin and fodrin, provides a physical structure to anchor secretory vesicles in proximity to the plasma membrane during epithelial cell secretion and exocytosis. A set of randomly organized MT, with their minus end and multiple MTOC embedded in a meshwork of cytokeratins, runs parallel to the apical (and basolateral) domain. Subapical IF also play an important role in maintaining cell integrity in digestive epithelia. Intestinal microvilli emerging from the apical membrane are supported by the apical actin meshwork and contain well-organized actin MF bundled by actin-binding proteins including villin, fimbrin (or plastin-1), and espin. However, the structural role play by actin-binding proteins has been recently questioned by the discovery that knockout mice lacking villin, fimbrin, and espin still produced microvilli, but have deficient retention of apical proteins into microvilli of enterocytes. The basolateral membrane is also lined by a meshwork composed of cross-linked arrays of actin. Focal adhesions connect this actin meshwork to the extra cellular matrix through integrins and their associated cytosolic actin-binding proteins. In addition, in the small intestine, keratin-associated proteins interact with desmosomes to establish cell-cell interactions. MT and their motors (dyneins and kinesins) are important in vesicle trafficking, in addition to organizing and positioning various organelles, such as Golgi, endosomes, and lysosomes. MF also contribute to scaffolding and motility of cytoplasmic components, providing also tracks for the myosin motor proteins involved in vesicle trafficking.
45.2.3
Membrane Compartments in Epithelial Cells
Polarized sorting operations largely occur within the Golgi and endosomes. These membrane organelles have a characteristic subcellular distribution. The Golgi, the chief biosynthetic sorting station, is located in the apical pole in close proximity to the nucleus, with tubular structures of the trans-Golgi network (TGN) projecting further toward to apex of the cell. Between the TGN and the apical membrane, an array of small membrane compartments or endosomes constitutes other key sorting stations. These compartments are generally named after their primary function. Apical early endosomes or basolateral early endosomes (AEEs or BEEs, respectively) are a heterogeneous population of endosomes that localize immediately under their respective membranes, have moderately acid internal pH, and contain the small GTPase Rab5. Common (recycling) endosomes (CEs or CREs), termed also subapical compartment (SAC) in hepatocytes, are marked by the small GTP-binding protein Rab8. In fully differentiated epithelial cells, a subapical cluster of membrane tubules is identified by the presence of Rab11 and named apical recycling endosome (ARE). Finally, late endosomes (LE) contain Rab7. A detailed description of the specific function of each compartment in protein trafficking is provided in Section 45.3 and is summarized in Fig. 45.2 .

45.3
Sorting Pathways
Newly synthesized proteins can travel via many different routes to reach their final apical or basolateral membrane destination. By maintaining multiple polarized trafficking routes, which can overlap with endocytotic sorting and recycling pathways, epithelial cells attain a fast and precise mechanism to regulate physiologically the location and density of cell surface proteins. For sake of simplicity, we first describe the three main biosynthetic pathways used for trafficking from Golgi to the apical and basolateral membranes, followed by a description of the postendocytic pathways. Both types share multiple compartments, as shown in Fig. 45.2 .
45.3.1
Direct Delivery
Newly synthesized proteins can follow a direct route from the TGN to their final apical or basolateral membrane destination ( Fig. 45.2 ). In this scenario, the apical and basolateral membrane proteins are sorted soon after their synthesis. The TGN has been classically considered to be the main sorting station of the biosynthetic pathway in epithelial cells, but it is becoming evident that sorting events in this route can also take place at various endosomal sorting compartments. In addition to sorting mechanisms, which segregate apical proteins from the basolateral membrane proteins before they reach their final destinations, other mechanisms are involved in the direct delivery pathway, including directional transport and specific docking of vesicle carriers with the appropriate membrane domains. Once delivered to the proper membrane, domain-specific retention may also contribute to maintaining appropriate polarity and cell surface density of specific membrane proteins.
45.3.2
Indirect Pathway
Steady-state polarity may also arise by a circuitous routing process, often called the indirect trafficking pathway. In this case, newly synthesized proteins are initially targeted to one plasma membrane domain and then either selectively retained or selectively retrieved and resorted to the opposite membrane domain. Such a pathway is favored for many apical membrane proteins in hepatocytes. In these cells, the majority of membrane proteins are first targeted from the TGN to the basolateral membrane, and then apical membrane-destined proteins are rerouted to the apical membrane by a process called transcytosis. Transcytosis is not limited to hepatocytes; in MDCK cells, subsets of newly synthesized proteins, including some glycosylphosphatidylinositol-anchored (GPI-anchored) proteins, are delivered first to the basolateral membrane and then, following a transcytotic route, are redirected to the apical membrane. Similarly, the polymeric IgA receptor (pIgR) is endocytosed from the basolateral membrane of MDCK cells and routed to the apical membrane via recognition of a specific transcytosis signal. As shown in Fig. 45.2 , the pIgR transcytotic route appears to involve sorting steps at BEE, CRE, and ARE, before being delivered to the apical membrane.
45.3.3
Random Pathway
Finally, newly synthesized membrane proteins can be randomly targeted to both plasma membrane domains and then be selectively retained or degraded, so that polarized sorting is achieved at the plasmalemma itself. For example, the alpha2B-adrenergic receptor has been reported to be delivered to both the apical and basolateral membranes of MDCK cells, but then are selectively retained exclusively at the basolateral membrane. Postendocytic pathway may also play an important role in the random pathway by selectively recycling specific proteins to the proper polarized membrane.
It is important to consider that protein-sorting pathways can be both cell- and protein-specific. For example, the intestinal brush border protein lactase-phlorizin hydrolase (LPH) is directly delivered to the apical surface of Caco-2 cells, while the human neurotrophin receptor p75 reaches its final apical destination via a basolateral transcytotic pathway. Certain pathways may predominate in a certain cell type, but less-traveled routes may be taken, depending on the specific protein. For example, while hepatocytes seem to favor the indirect pathway, canalicular (apical) ABC (ATP-binding cassette) transporters MDR1, MDR2 (multidrug resistance proteins 1 and 2), and SPGS (sister of p-glycoprotein) have been shown to follow a direct biosynthetic delivery pathway. In some cases, the type of epithelial cell defines the route taken by a specific protein. For example, gp80 is sorted to the basolateral membrane of T84 human colonic adenocarcinoma cells, but is expressed on the apical plasma membrane of MDCK cells. In other instances, the same protein may be targeted to identical destinations regardless of the epithelial cell type, albeit through completely different trafficking pathways. Such is the case with the human neurotrophin receptor p75, which follows an indirect route to the apical membrane of intestinal Caco-2 cells, but is directly routed to the apical membrane in MDCK cells.
45.3.4
Protein Sorting in the Biosynthetic Pathway
45.3.4.1
Discovery
Pioneering studies describing direct apical or basolateral sorting pathways took advantage of the ability of MDCK cells to generate well-polarized monolayers when grown on permeable supports, and observations that certain viruses emerge from infected MDCK cells in a polarized manner. For example, the influenza virus buds exclusively from the apical membrane, while the vesicular stomatitis virus (VSV) emerges only from the basolateral membrane. This asymmetric budding response is a consequence of polarized expression of viral coat proteins and has provided an extremely powerful tool to elucidate the mechanisms of asymmetric sorting processes in epithelial cells. Of special interest, the influenza hemagglutinin coat protein (HA) accumulates on the apical membrane, as opposed to the VSV G coat protein, which localizes exclusively to the basolateral membrane. Studies performed using plasmalemma domain-selective detection techniques showed that newly synthesized HA and VSV G are directly delivered to their target membranes without any transitory stops on opposite plasma membrane domains. A direct trafficking route indicates that proteins must be sorted within an intracellular compartment, prior to vectorial delivery to their target membranes. This trafficking pathway was found to be also common for many endogenously expressed proteins, as reported by studies employing a cell-surface biotinylation technique in various cultured cell lines.
45.3.4.2
Golgi is a Key Biosynthetic Sorting Station
Immunoelectron microscopic examination of doubly infected MDCK cells showed that both HA and VSV G glycoproteins traversed the Golgi apparatus and even the same Golgi cisternae. Thus, critical segregation steps were thought to take place somewhere between the end of Golgi and the plasma membrane. Support for segregation within the TGN came from the observations that traffic of both apical and basolateral membrane proteins can be arrested at the trans-Golgi by incubation at 19–20°C. Upon release from block, viral membrane proteins proceed rapidly and directly to their appropriate apical or basolateral plasma membranes, without obligate stops in endosomes or other intracellular compartments. Moreover, following low-temperature Golgi block and release, TGN vesicles containing either apical or basolateral cargo could be separated using equilibrium density gradient centrifugation combined with immunoisolation techniques, providing evidence of a sorting event at the TGN. High-voltage electron microscopy studies combined with computer axial tomography provided evidence for distinct coat-based sorting domains in the TGN and showed that the TGN consists of multiple tubules, containing clathrin or novel “lace-like” coats.
Additional evidence of the apical and basolateral membrane protein sorting at the TGN was obtained using time-lapse fluorescence imaging in live cells transfected or infected with basolateral and apical membrane marker proteins. The apical and basolateral membrane cargo progressively segregate within domains of the Golgi and TGN, exclude resident proteins, and then exit in separate tubulovesicular carriers in direct route to the plasmalemma. Budding and fission of tubulovesicular carriers from the TGN is controlled by dynamins or dynamin-related large GTPases. Interestingly, dynamin-2 appears to be involved only in fission of tubulovesicular carriers containing the apical marker protein p75, while the dynamin-related carboxy terminal-binding protein 3/brefeldin A-ribosylated substrate (CtBP3/BARS) is specific for tubulovesicular carriers of the basolateral marker VSV G. Tubulovesicular carriers destined for either the apical or the basolateral membrane also differ in their composition. A recent study in MDCK cells showed that exit from TGN of many basolateral proteins was impaired by clathrin knockdown, while exit of apical proteins was unaffected. Interestingly, basolateral membrane-directed trafficking of the Na,K-ATPase beta subunit was also independent from clathrin, consistent with the existence of an alternative basolateral membrane sorting mechanism.
45.3.4.3
Other Sorting Stations for Newly Synthesized Proteins
Some apical and basolateral proteins may be marked for sorting an earlier step in the biosynthetic pathway. Routing the proteoglycan serglycin provides an example. Serglycin is targeted to either the apical or the basolateral membrane in MDCK cells, depending on the chemistry of the glycosaminoglycan side chains; glycoproteins destined to the basolateral surface of MDCK cells are shorter and more intensely sulfated than their apical counterparts. In addition, the basolateral membrane-destined seglycin molecules contain more heavily sulfated chondroitin sulfate chains than those directed to the apical membrane. Because these modifications take place before delivery to the TGN, it has been argued that sorting must take place earlier in the biosynthetic pathway. Furthermore, serglycin has been shown to traffic to the apical membrane in the presence of the Golgi transport-blocking drug brefeldin. In another example, the oligomerization of apical GPI-anchored proteins (GPI-APs) that takes place in rafts or detergent-resistant microdomains (DRMs) within the cis-Golgi is a critical step for their sorting. Paladino et al. showed that basolateral GPI-APs were also associated to DRMs in the Golgi, but did not form high molecular weight complexes. As a result, inhibition of raft formation by depleting cells of cholesterol affected sorting of apical, but not basolateral, GPI-APs. Furthermore, appropriate cholesterol-dependent oligomerization at the Golgi was recently found to be an important function to achieve appropriate organization and function of GPI-APs at the apical membrane in MDCK cells.
Polarized trafficking from the TGN to the plasma membrane domains has been shown to follow a direct path, but it should be pointed out that sequential intracellular routing steps, involving intermediate endosomal compartments, have been identified. Orzech et al. implemented an assay to measure the meeting of newly synthesized membrane proteins with endosomal compartments loaded with horseradish peroxidase, and found that the biosynthetic road traveled by polymeric immunoglobulin receptors can involve an endosomal compartment, most likely the common recycling endosome. Because apical and basolateral proteins intermix in this compartment (see below), it is likely that the CRE might also function as a polarized sorting station for some proteins in the biosynthetic pathway.
45.4
Postendocytic Pathway
Since nearly 50% of a typical polarized plasma membrane is endocytosed per hour, specific sorting mechanisms must be in place to ensure that endocytosed proteins are recycled back to the appropriate plasma membrane. In addition, sorting in the postendocytic pathway is also important for polarized distribution of proteins that are processed in indirect pathway by transcytosis and for the general maintenance of epithelial cell polarity. Indeed, constant internalization and sorting in the postendocytic pathway may offer a continuous surveillance or proofreading mechanism to relocate proteins that have randomly been sorted or missorted in the biosynthetic pathway. Furthermore, since sorting in the biosynthetic pathway is only a one-time event in the lifetime of a protein, continuous postendocytic sorting is quantitatively more important.
Study of the intracellular trafficking itineraries of endocytotic recycling (e.g., transferrin and its receptor, TfR) and transcytotic marker proteins (e.g., IgA and its receptor, pIgR) in model epithelia, such as MDCK cells and CaCO-2 intestinal cells, has shown at least four distinct populations of endosomal sorting compartments (see Fig. 45.2 ). Although more complex in epithelial cells, these endocytic compartments are similar to early and recycling endosomes found in nonpolarized cells.
Internalization of fluid-phase markers from the basolateral or apical surface of MDCK cells revealed that material internalized from opposite membrane domains first accumulates in spatially distinct populations of early endosomes, described as BEEs and AEEs. The AEE and BEE appear to be biochemically and physiologically distinct from each other, despite the presence of common biochemical markers, like Rab 4, Rab 5, and the Rab effector EEAI. Both endosomal compartments exhibit different overall protein compositions, recycling rates, fusigenic properties, and dependence on filamentous actin. Therefore, it appears that each type of early endosome is designed to support rapid and efficient membrane protein recycling to a specific plasma membrane domain, preventing mixing of the apical and basolateral membrane proteins. Material endocytosed into AEE or BEE can be rapidly recycled without passing through intermediated compartments, a process dependent on Rab 4. In some cases, such as with PDGFβ, recycling through these early endosomes is regulated. Alternatively, proteins that are not immediately recycled may be targeted into the lysosomal pathway or directed to a common recycling endosome (reviewed in Ref. ).
The CRE receives and recycles endocytosed material from both the apical and basolateral membranes and it is considered to be a major polarized sorting station (see Figs. 45.2 and 45.3 ). It is generally identified by a perinuclear localization and a tubular morphology, the presence of Rab 11 and/or Rab 17, and the absence of fluid phase markers. Rab 8 has been reported to identify a subset of CRE that are involved in basolateral sorting in kidney cells, but this compartment serves as a platform to control apical protein localization in intestinal cells, perhaps via an apical-membrane generation complex. Because of its clearly differentiated composition, the CRE from MDCK cells can be resolved from early endosomes physically, biochemically, and pharmacologically. Ultrastructural studies showed that basolateral sorting of the transferrin receptor takes place in tubular extensions of the CRE on clathrin-studded vesicles. Similar to the biosynthetic pathway, the sorting process in the CRE is sensitive to Brefeldin A (BFA). The BFA is believed to alter endosomal-sorting processes directly by inhibiting the association of adaptors and coat proteins (see Section 45.6.1.1 ) by Arf proteins, which in turn prevents critical segregation steps in the CRE.

Endocytosed apical proteins may also be transferred from AEE to another specialized compartment, the ARE, also named the SAC in hepatocytes. The ARE is likely to serve as a depot for membrane proteins traveling between the apical membrane and CRE (reviewed in Ref. see Fig. 45.2 ), and has received much attention because it is suspected to be a postendocytic hub where apical membrane traffic is regulated to control apical membrane protein surface density. It should be noted that there has been some debate whether ARE is actually a separate endosomal entity or subdomains of CRE. Efforts to differentiate between both compartments have been hampered because of their overlapping or common properties. Both ARE and CRE compartments have a subapical, perinuclear, or pericentriolar cellular location, lack fluid-phase markers, are dependent on MT, and present a similar set of Rab proteins. Later studies employing confocal microscopy or studying the segregation of co-internalized marker proteins and fluid-phase markers provided several criteria for distinguishing ARE from the CRE in MDCK cells. Brown defined the ARE as an endocytic compartment at the extreme apical pole that is enriched in apical proteins. The CRE, in contrast, has an apical and lateral distribution in the cell cytoplasm and contains apical and basolateral proteins in equal proportion. Confocal microscopy studies showed that Rab 11a predominately associates with the apical-membrane protein-enriched vesicles at the extreme apical pole rather than with the CRE. Furthermore, the CRE is TfR-rich, while the ARE contains myosin Vb and lacks rapidly recycling TfR. In addition, differences in the intravesicular pH of the CRE (acidic) and ARE (neutral) provides a functional dissimilarity, which can be exploited to differentiate between these two compartments.
Whether sorting occurs in the TGN or CRE, it generally involves segregation of cargo and the assembly of trafficking vesicles by coat proteins and adaptor molecules or complexes. Selective attachment of a specific coat factor to cargo is believed to promote segregation and concentration of select cargo while specifically marking vesicle carriers for the apical or basolateral membrane. However, selective partitioning in specialized lipid domains may provide an alternative mechanism for sorting of apical proteins (see Section 45.3.3 ).
45.5
Sorting Signals
Polarized sorting is directed by signals embedded within the structure of polarized membrane proteins. They are interpreted and acted on by intracellular sorting machineries to segregate, initiate vesicle formation, transport, or retain target proteins on appropriate membrane domains. A great deal of research activity has focused on identifying these signals, usually through mutagenesis and peptide-motif transplantation studies. By definition, polarized expression is lost when a sorting signal is mutated. However, different missorting phenotypes may be observed. In some cases, proteins can exhibit a complete loss of polarity, and become randomly localized to the apical and basolateral membranes. In contrast, when dominant signals are deleted and effects of recessive sorting signals are manifested, mutant proteins will be mistargeted to the opposite membrane domain as the wild type. Although mutagenesis studies provide evidence that a structure is necessary for sorting, it is important to demonstrate that the structure is also sufficient for polarized sorting. In this case, the signal should be able to act in an autonomous fashion and confer a polarized sorting phenotype to a reporter protein that otherwise lacks sorting signals.
45.5.1
Basolateral Sorting Signals
Sorting of basolateral proteins is typically defined by small amino acid motifs within their cytoplasmic domains. Two different classes of signals have been identified. One class, similar to clathrin-dependent endocytic or lysosomal sorting signals (reviewed in Ref. ), includes short tyrosine-containing motifs NPXY or YXXΦ, where Φ is a hydrophobic residue, and is found, for example, in the LDL receptor proximal signal or in the VSV-G protein. In addition, dileucine/dihydrophobic signals fitting the consensus motif D/EXXLL have been identified in, for example, the IgFc receptor, the MHC, class II protein, E-cadherin, and NKCC1. Furthermore, a related basolateral sorting signal featuring only one leucine residue has been identified in amphiregulin (EEXXXL). The similarity of these signals with endocytic motifs, their direct interaction with clathrin-adaptor molecules (see Section 45.6.1 ), and more recently the role of clathrin itself in basolateral sorting suggest that both basolateral sorting and endocytosis share some common elements.
Other basolateral sorting signals do not share any homology to endocytic signaling motifs and likely represent several distinct kinds of unrelated sorting signals. These include the H/RXXV motif in the polymeric immunoglobulin receptor, pIgR, which has been proposed to form a beta-turn structure that interacts with BFA-sensitive adaptor complexes to direct basolateral sorting. Some bipartite basolateral sorting motifs include the acidic cluster motifs that are either juxtaposed to critical tyrosine residues (e.g., the LDL receptor distal determinant ) or to di-hydrophobic residues (e.g., in furin, and the stem cell factor ). Intriguingly, two basolateral sorting signals composed of small acidic clusters followed by proline or hydrophobic residues at variable distances have been found in the proton-coupled monocarboxylate transporter MCT3 and MCT4, and promote clathrin-dependent, but clathrin-adaptor AP1B-independent, basolateral sorting. Finally, other basolateral sorting signals are juxtaposed to PDZ-binding motifs at the extreme carboxyl terminus, like those in the betaine transporters, the Kir 2.3 channel and the GAT-2 GABA transporter, although these signals appear to operate independent of the PDZ ligand.
45.5.2
Apical Sorting Signals
Apical sorting signals are diverse in their nature and distribution, since their composition may include specific amino acid motifs, or lipid or carbohydrate modifications. Furthermore, they can be localized to the extracellular, transmembrane, or cytosolic domain. These signals are often associated with membrane domains and structures that determine clustering, control glycosylation, or dictate membrane microdomains association. For instance, specific GPI anchors allow selective partitioning of some apical proteins into lipid rafts at the Golgi apparatus. Interestingly, GPI anchors appear to be necessary, but not sufficient, to ensure apical sorting (see Section 45.6.3 ). In other cases, N- and O-linked glycosylation appears to be required for apical delivery. For example, deletion of one N-glycan chain from erythropoietin resulted in nonpolarized distribution, while removal of O-glycans from neurotrophin receptor results in missorting to the basolateral membrane. Conversely, insertion of an N-glycosylation signal in the nonglycoprotein rat growth hormone resulted in apical secretion, rather than secretion without polarity. It should be noted that in many cases, removal of N-glucans results in retention at the endoplasmic reticulum, complicating interpretation toward a full understanding of the role of glycosylation in polarized sorting. To add further complexity, the composition of glycosaminoglycan chains also determines sorting, with chondroitin sulfate proteoglycans being preferentially sorted to at the apical membrane, and heparin sulfate proteoglycans being routed basolaterally. The importance of glycan groups in polarized protein sorting may reflect the requirement for proper cargo folding and/or the involvement of apical cargo receptor lectins, such as VIP36, galectin-3, 4, or 9, which may participate in protein sorting or raft-independent clustering events.
Another example of raft-independent targeting is provided by transmembrane and cytosolic apical sorting signals. A transmembrane domain and flanking regions in the gastric H + /K + ATPase α-subunit form a structure that is necessary to direct this transporter to the apical membrane. Some apical sorting determinants have recently been identified within the protein sequence of cytoplasmic domains of, for example, rhodopsin, Na-K-2Cl cotransporter type 2 (NKCC2), receptor guanylate cyclases, and ATP7B copper-ATPase. Interestingly, the endocytic receptor megalin contains apical sorting determinant that includes a NPXY-type motif, suggesting that apical sorting of some proteins may also involve clathrin-adaptor molecules.
45.6
Recognition of Sorting Signals
A great deal of research activity has focused on identifying and characterizing the intracellular machineries that directly interact with intracellular sorting signals and decode it. Proof that a sorting machinery candidate directly participates in the signal recognition event requires evidence that it directly interacts with a specific sorting signal, localizes to the appropriate sorting compartment, and is required for polarized sorting. Disruption of interaction with cargo in vivo, ideally specific mutation of the signal recognition site in the sorting machinery, should also produce the same cargo missorting phenotypes as when the sorting signal is mutated. While few of candidates meet all of these rigorous standards, insights can be gained by a review of our present understanding.
45.6.1
Clathrin Adaptors
The similarity of tyrosine- and dileucine-based basolateral sorting signals to those involved in clathrin-dependent endosomal and lysosomal targeting (reviewed in Ref. ) have long suggested the possibility that basolateral sorting may involve a mechanism similar to clathrin-adaptor-dependent internalization and endosomal trafficking. The discovery and characterization of μ1B, an epithelial-specific “medium” subunit of the clathrin-adaptor AP-1 complex, coupled with observations that clathrin is required for the sorting of many basolateral proteins in MDCK cells strongly suggest that this is, in fact, the case.
Heterotetrameric clathrin-adaptor complexes (AP), called adaptins (reviewed in Ref. ), interact with tyrosine- and dileucine-based motifs (see Fig. 45.3 ). Four adaptor complexes (AP-1 to AP-4) have been described. Each adaptor is composed of two large subunits (γ/β1, in AP-1; α/β2, AP-2; δ/β3, AP-3; ε/β4, AP-4), one medium subunit (μ1–4), and one small subunit (σ1–4). Tyrosine-based “YXXΦ” motifs interact with the medium μ1–4 subunits, within a structurally defined binding pocket. The smaller σ subunit and the α subunit harbor the binding site for dileucine “[D/E]XXXL[L/I]” motifs in AP-2. Both signal recognition sites are located within the trunk domain of the adaptor complex, far removed from the clathrin-binding domains in the hinge region of AP-1 and AP-2. Consequently, AP-1 and AP-2 adaptor complexes have the capacity to interact simultaneously with clathrin and membrane proteins containing tyrosine- or dileucine-based signals. In this way, AP-1 and AP-2 couple cargo recognition to clathrin coat formation. AP-3 and AP-4 do not interact with clathrin directly, and it remains unknown if and how these adaptors control vesicle formation.
Each AP complex specifies a distinct trafficking operation (see Fig. 45.4 ). The AP-1A complex participates in the delivery of cargo from the trans-Golgi to the endosomal-lysosomal system and the basolateral membrane; AP-1B controls basolateral trafficking from the recycling endosome, and, possibly, the TGN; AP-2 mediates endocytosis of membrane proteins, AP-3 has been implicated in endosomal and lysosomal delivery, AP-4 has been associated with Golgi to endosome transport and basolateral traffic. Targeting specificity of the different adaptor complexes is governed, at least in part, by their different affinities for different sorting compartments, which is determined by their different phosphoinositide-binding properties. Different adaptins also exhibit somewhat different cargo-binding properties. The types of YXXΦ and [D/E]XXXL[L/I] signals that are recognized by each adaptor complex overlaps to a significant extent, but each AP complex exhibits differing preferences for residues neighboring the critical tyrosine residue and the dileucine motif.

45.6.1.1
AP-1B
Sorting of many, but not all, basolateral proteins require AP-1B complex (γ/σ1/β1/μ1B). Distinguished by the presence of its epithelial specific μ1B subunit, the AP-1B is biochemically and spatially distinct from the ubiquitous AP-1A adaptor (γ/σ1/β1/μ1A). It mainly localizes to the CRE, although some studies have suggested that it also localized to the TGN. From these compartments, AP-1A marks proteins traveling in the biosynthetic and postendocytic pathways for delivery to the basolateral membrane. The discovery evolved from elegant studies that made use of unusual properties of a pig kidney epithelial cell line, LLC-PK1. LLC-PK1 cells lack AP-1B and “mistarget” proteins containing tyrosine-based basolateral sorting signals to the apical membrane. When μ1B subunits are stably expressed in LLC-PK1cells, however, they co-assemble into heterotetrameric AP-1B complexes, and allow basolateral sorting of target proteins that are otherwise apically expressed in wild-type LLC-PK1 cells.
The most likely explanation for the requirement of AP-1B is that it directly interacts with tyrosine-based sorting signals, recruiting proteins containing them into clathrin-coated vesicles (CCVs) for the transport to the basolateral membrane. Interestingly, many of the signals recognized by AP-1B, such as the one in the LDLR, do not conform to the canonical “YXXΦ” adaptin-binding motif. Other proteins, such as the TfR, require μ1B for their sorting but rely on sorting signals that share no resemblance to any known clathrin-adaptor-binding motif (see Section 45.5 ). Some of these signals may interact with μ1B through a binding site other than that used by YXXΦ motifs. In support of this idea, mutant μ1B subunits rendered defective for YXXΦ binding are unable to support the basolateral membrane targeting of proteins containing such motif, but are still able to the support basolateral membrane expression of LDLR and TfR.
The existence of alternative routes to the basolateral membrane that are completely independent of AP-1B or even clathrin should be emphasized. For example, the IgG Fc receptor FcR11-B2, the Kir 2.3 channel, and the Na,K-ATPase depend on alternative but still uncharacterized basolateral sorting mechanism. The FcR11-B2 contains a dileucine-type basolateral targeting signal, which presumably has the capacity to interact with α-adaptin subunits. Thus, other adaptin complexes (such as AP-4, see below) or adaptin-like molecules may also be involved in the basolateral targeting of some of these proteins.
45.6.1.2
AP-4
The adaptor protein AP-4 is expressed in both polarized and nonpolarized cells, where it localizes to the TGN and endosomes. It has been found to interact in vitro with the basolateral sorting signals of furin, LDLR, and the TfR. Importantly, RNA antisense-mediated studies revealed that the μ4 subunit in MDCK cells is required for basolateral sorting of LDLR and furin, but not transferrin. Thus, it is the likely candidate to mediate recruitment of cargo for basolateral trafficking in a clathrin-independent and an AP-1B-independent sorting pathway (see Fig. 45.4 ). More recent studies with the AP-4 routing-dependent Alzheimer disease-related amyloid precursor protein (APP) raise the possibility that AP-4 might mediate basolateral delivery through a TGN-to-endosome routing pathway.
45.6.2
Vesicle Formation and Budding
Sorting signal recognition is often coupled to vesicle formation. Coated vesicles have a critical role in concentrating, packaging, and shuttling cargo between different intracellular compartments and plasma membrane domains (reviewed in Refs. ). Three different types of coated vesicles have been described based on their compositions and intracellular compartments where they originate. The CCVs mediate transport between TGN, endosomes, and the plasma membrane. Coatomer complex I (COPI) and coatomer complex II (COPII) coated vesicles mediate intra-Golgi or Golgi to ER retrograde transport, and ER to Golgi transport, respectively. Formation of all the three types of coated vesicles shares a common sequential mechanism, whereby coat subunits assemble on the membrane and recognize cargo. As cargo and coat proteins concentrate, the underlying membrane deforms, buds from the parent membrane and is cut off, forming a coated vesicle.
The three types of coated vesicles recognize specific sets of cargo and have different protein and lipid compositions. The CCVs are composed of two cytosolic protein complexes: clathrin triskelia and heterotetrameric adaptor complexes (AP-1 and AP-2). The COPII vesicles have a similar structure, but the two protein complexes are Sec13-31 and Sec23-24. The COPI coat is somewhat more simple and contains a single multisubunit coatomer complex. Despite their differences, all these protein complexes have a common tendency to self-assemble into empty spherical cages, similar to the outer cage found in vesicles in vivo. Cage subunits cannot bind directly to the membrane, and require an inner adaptor layer capable of simultaneous interaction with cage protein complexes, sorting motifs in cargo proteins, and the compartment membrane via negatively charged lipids.
Sculpting the membrane into the curved shape of a vesicle is an energy-intensive process that requires recruitment of additional proteins. Two related GTPases, Arf1 in COPI vesicles, and Sar1 in COPII vesicles, appear to manage both coat recruitment and curvature generation. A similar role is played by the GTPase dynamin in CCV, although additional proteins such as amphiphysin, epsin, endophilin, and members of the sorting nexin family are also recruited and play a role in binding to members of the core component, or recognizing, generating, and stabilizing the membrane curvature. The GDP-bound Arf1 associates with the membrane, and upon GTP binding it undergoes a conformational change. This change exposes a short amphipathic N-terminal helix that gets inserted into the lipid bilayer, causing an asymmetric expansion of the outer versus the inner leaflet, allowing Arf1 to remodel the membrane into highly curved structures.
Similar to Arf1, Sar1 action also follow a cycle of GTP binding, followed by association to the membrane, recruitment of the adaptor complex Sec23-24, and a conformational change that induces curvature of the membrane. Formation of CCVs appears to be more complex, with multiple accessory proteins interacting with the membrane through structural elements such as BAR (Bin, amphiphysin, Rvs) or ENTH (epsin N-terminal homology) domains. In addition, dynamin contains a PH domain that provides docking sites for negatively charged lipid headgroups. The GTP-bound dynamin readily associates with the membrane and inserts its PH domain in the lipid bilayer, inducing curvature of the membrane. The scaffolding role of dynamin allows this protein to interact with multiple SH3-domain containing accessory proteins, which in turn contribute to stabilize the membrane curvature. Finally, dynamin has also been shown to play an important role in vesicle scission and release from the donor membrane, a process also known to require GTP hydrolysis.
45.6.3
Apical Routing Via Lipid Rafts and Associated Molecules
Sorting signals may also govern lipid partitioning into membrane domains, like “rafts.” In fact, lipid rafts were first defined as membrane microdomains rich in glycosphingolipids and cholesterol, which serve as sorting platforms and vectorial delivery vehicles for apical membrane proteins and lipids traveling in the biosynthetic pathway. In support of this model for apical trafficking, nearly all endogenous GPI-APs expressed in MDCK were apically localized due to their incorporation into detergent-resistant membrane (DRM) domains as they reach the Golgi complex. In addition, depletion of glycosphingolipids or cholesterol results in missorting of apical GPI-APs. The correlation between GPI-linkage, DRM association, and apical membrane delivery suggested that GPI anchors might serve as raft-dependent apical sorting signals. This view was initially reinforced by the observation that chimeric GPI-APs are directed to the apical membrane.
The results of these studies were challenged by the observation that in Fisher rat thyroid cells the majority of endogenous GPI-APs are delivered to the basolateral membrane, while in hepatocytes they are fist targeted to the basolateral membrane and then transcytosed to the apical domain. Furthermore, in MDCK cells not all lipid raft-associated proteins are apical or affected by cholesterol depletion. Moreover, in MDCK cells some GPI-APs are also delivered first to the basolateral membrane and then moved to the apical surface by transcytosis.
The controversy of whether glycosylphosphatidylinositol anchors (GPI anchors) are true apical sorting signals is beginning to be clarified by studies showing that not all GPI anchors are the same, and by the realization that raft association is a more complex process than originally thought. Both the apical and basolateral GPI-APs associate with DRMs, but only apical GPI-APs cluster into large oligomers that are selectively routed to the apical membrane. A mechanistic understanding of this critical clustering event has been provided in a recent work by Kinoshita et al. These investigators found that the unsaturated fatty-acid chains present in GPI anchors at the ER are turned into saturated fatty-acid chains as they travel through Golgi by specialized Golgi-resident enzymes. This fatty-acid maturation is critical for GPI-APs association into lipid rafts. Variations in the sensitivity of individual GPI anchors to these enzymes condition their final inclusion into lipid rafts, and, consequently, determine whether they are targeted to the apical or basolateral membrane.
The GPI anchors are the most common raft association signal, but other raft association mechanisms have also been described. For example, apical sorting determinants within transmembrane domains of hemagglutinin (HA) and neuraminidase are colinear with the structural determinants of DRM association. The disruption of DRM by cholesterol removal causes missorting of these proteins to the basolateral membrane, and DRM association is governed, at least in part, by their transmembrane apical sorting signals.
The association with raft-associated molecules also plays a role in targeting proteins for inclusion in lipid rafts. For example, in intestinal cells, Galectin 4 participates in lipid raft clustering via interaction with glycosphingolipids and N-linked complex carbohydrates in rafts-associated glycoproteins. Annexin II and annexin 13b are Ca 2 + -dependent lipid-binding proteins that associate with DRM and are required for raft-dependent trafficking of some apical membrane proteins. Annexin 13b directly interacts with an apical membrane-directed microtubule motor protein, KIFC3, suggesting a role in apical membrane-directed delivery of DRMs. Annexin II plays a role in the sorting and transport of brush border hydrolases, such as sucrase isomaltase, as well as structural components such as ezrin, and may even play a broader role in maintaining intestinal cell polarity. Downregulation of annexin II in Caco-2-A4 cells results in a severe reduction in the levels of the brush border membrane resident enzyme.
It should be pointed out that lipid microdomain association can play a role in basolateral polarity. Some basolateral membrane proteins also partition into specialized cholesterol-enriched compartments called caveolae, which are primarily found on the basolateral membrane in intestinal cells, native kidney, and MDCK cells.
45.6.4
Rab GTPases
The Rab GTPases regulate the spatial and temporal organization of numerous membrane trafficking processes, such as vesicle budding, targeting, and tethering as well as the docking and priming stages of vesicle fusion.
The Rab proteins appear to orchestrate trafficking events by specifically associating with a precise membrane compartment that contains a unique set of effector proteins (reviewed in Ref. ), making them ideal components of polarized sorting machines.
Several Rabs have been implicated in apical membrane sorting processes (see Fig. 45.4 ). The epithelial-specific Rabs, Rab17, and Rab 25, along with the more widely expressed Rab 11a, localize to apical endosomes. Of special interest, in gastric parietal cells Rab11a, along with Rab 25 and the SNARE proteins syntaxin 3 and VAMP2 (see Section 45.8.1 ) appear to be critical in promoting regulated apical membrane insertion of the H,K-ATPase responsible for acid secretion into the secretory canalicular membrane. Rab 17 has been implicated in the regulation of traffic through ARE in MDCK. More recently, Rab14 has been found to localize to the TGN and apical endosomes to regulate delivery of cargo to the apical domain.
Other Rabs, particularly Rab 8, have been implicated in basolateral membrane processing steps for proteins travelling in the μ1B adaptor-dependent pathway in MDCK cells, although surprisingly, the same Rab has been found to regulate apical membrane localization in intestinal cells. In addition, Rab10 has been proposed to participate in basolateral targeting form CRE.
Interestingly, Rab3b has been found to interact with a specific 14 amino-acid site in the cytoplasmic domain of the pIgR receptor that was previously shown to be required for transcytosis. Regulated dissociation of Rab3b from pIgR stimulates basolateral to apical pIgR transcytosis, presumably by triggering translocation of receptor-containing vesicles from the ARE to the apical membrane. In this way, direct interaction of vesicle cargo with an Rab can determine its own intracellular traffic. A direct cargo interaction mechanism may have evolved for very abundant membrane proteins, like pIgR, to provide a dedicated high fidelity and robust trafficking mechanism.
45.7
Polarized Transport and Delivery
For polarized protein expression to be achieved, transport carriers must be correctly delivered to the appropriate acceptor membrane. Current evidence indicates that long-range transport may be carried out by MT while short-range transport near the cell surface is carried out by actin. In addition, specific sets of molecular motors appear to be involved in apical and basolateral trafficking.
45.7.1
Microtubule-Mediated Transport
The unique organization of MT in polarized epithelial cells (see Section 45.2.2 ), along with the presence of directional MT-motor proteins, has long suggested an important role for MT in apical and basolateral membrane transport. The MT have been shown to facilitate the rapid (~ 0.1–1 μm/s) and efficient movement of vesicles to the plasmalemma (reviewed in Ref. ). Apical traffic is generally more sensitive to microtubule disruption with agents such as nocodazole or colchicine than basolateral traffic. The MT disruption affects delivery of proteins to the apical membrane irrespective of whether they are traveling in the biosynthetic, the apical recycling, or the transcytotic pathways. Basolateral trafficking is more resistant to MT disruption, with some proteins being more sensitive than others. More recently, similar conclusions have been reached using real-time imaging techniques to directly monitor the role of MT in trafficking and fusion of post-Golgi transport intermediates, carrying apical- or basolateral-destined proteins.
The MT motors are remarkably selective, associating with select vesicles to ensure movement in the appropriate direction. In some cases, MT motors may associate with a transport vesicle by directly interacting with the cargo proteins. For example, the dynein light chain directly interacts with an apical sorting signal in the COOH-terminal tail of rhodopsin to mediate trafficking from TGN to the apical membrane. Sometimes, transport of one cargo may require sequential intervention of two different motor proteins at different steps. For example, the apical marker, p75, requires dynein for release of vesicles from the TGN, and kinesin for the formation and transport of apically directed tubulovesicular carriers.
The minus-end-directed motors, such as dynein and the unconventional kinesins, selectively carry vesicles toward the apical membrane. Conventional kinesin motor proteins are plus-end-directed motors and transport vesicles to the basolateral membrane, as well as along the mixed polarity subapical MT network. For example, in the renal collecting duct, dynein participates in the transport of AQP2 water channels to the apical membrane. Trafficking of the human sodium-dependent multivitamin transporter (hSMVT) in intestinal cells appears to involve distinct trafficking vesicles that require an intact MT network and the motor protein dynein for their mobility. KIFC3, a minus-end-directed kinesin, transports HA and annexin XIIIb to the apical membrane. In contrast, the plus-end microtubule molecular motor kinesin-1 participates in traffic of the Na,K-ATPase to the basolateral membrane of alveolar cells.
Intriguingly, conventional plus-end-directed kinesin motors (KIF5 family) have also been shown to support traffic of vesicles to the apical membrane, likely by using a recently discovered highly dynamic population of MT that preferentially grow their plus ends toward the apical pole. For example, KIF5B supports TGN exit of the apical marker p75, and KIF5C mediates a similar role for both the raft-associated sucrase isomaltase and the raft-independent neurotrophin receptor.
45.7.2
Actin-Mediated Transport
Transport along actin filaments, slower than transport along MT, is driven by myosin motor proteins which, except for myosin VI, move toward the barbed (+) end of actin filaments. Studies on myosin motors have provide insight into the role of the actin cytoskeleton in polarized trafficking. For example, myosin Vb interacts with Rab 11 in CRE and is involved in traffic between the ARE and the apical surface. Myosin I has been suggested to play a role in raft-associated apical traffic of sucrase-isomaltase in intestinal cells. Myosin motors have also been shown to play significant roles in basolateral traffic. For example, myosin IIa is found at the Golgi apparatus and has been shown to promote selective exit of basolateral proteins. Myosin VI has been shown to be required for sorting of basolateral cargo in the m1B-dependent pathway in MDCK cells. In addition, Myosin-Vc is expressed primarily in epithelial cells and interacts with Rab 8, suggesting a possible similar role in a basolateral m1B-dependent sorting pathway.
45.8
Polarized Docking and Fusion
Specific molecular machinery provides the force required for the fusion of donor and acceptor membranes, exerting a critical control to ensure that an individual vesicle fuses with the right target membrane, providing a final “proof reading” step in the polarized protein trafficking process.
45.8.1
v-SNAREs and t-SNAREs
The core machinery for vesicle fusion is composed of a family of structurally related integral membrane proteins, known as soluble N-ethyl maleimide-sensitive attachment factor receptors (SNAREs), and two soluble proteins named N-ethyl maleimide-sensitive factor (NSF) and soluble NSF attachment factor (α-SNAP). The initial step in this membrane fusion event involves the interaction in cis of two SNAREs at the target membrane (t-SNAREs), generally homologs of the syntaxin and/or SNAP-25. The resulting heterodimeric complex then interacts in trans with a vesicle SNARE (v-SNARE), generally a VAMP/synaptobrevin homologue, to form a fusion complex (see Fig. 45.5 ).

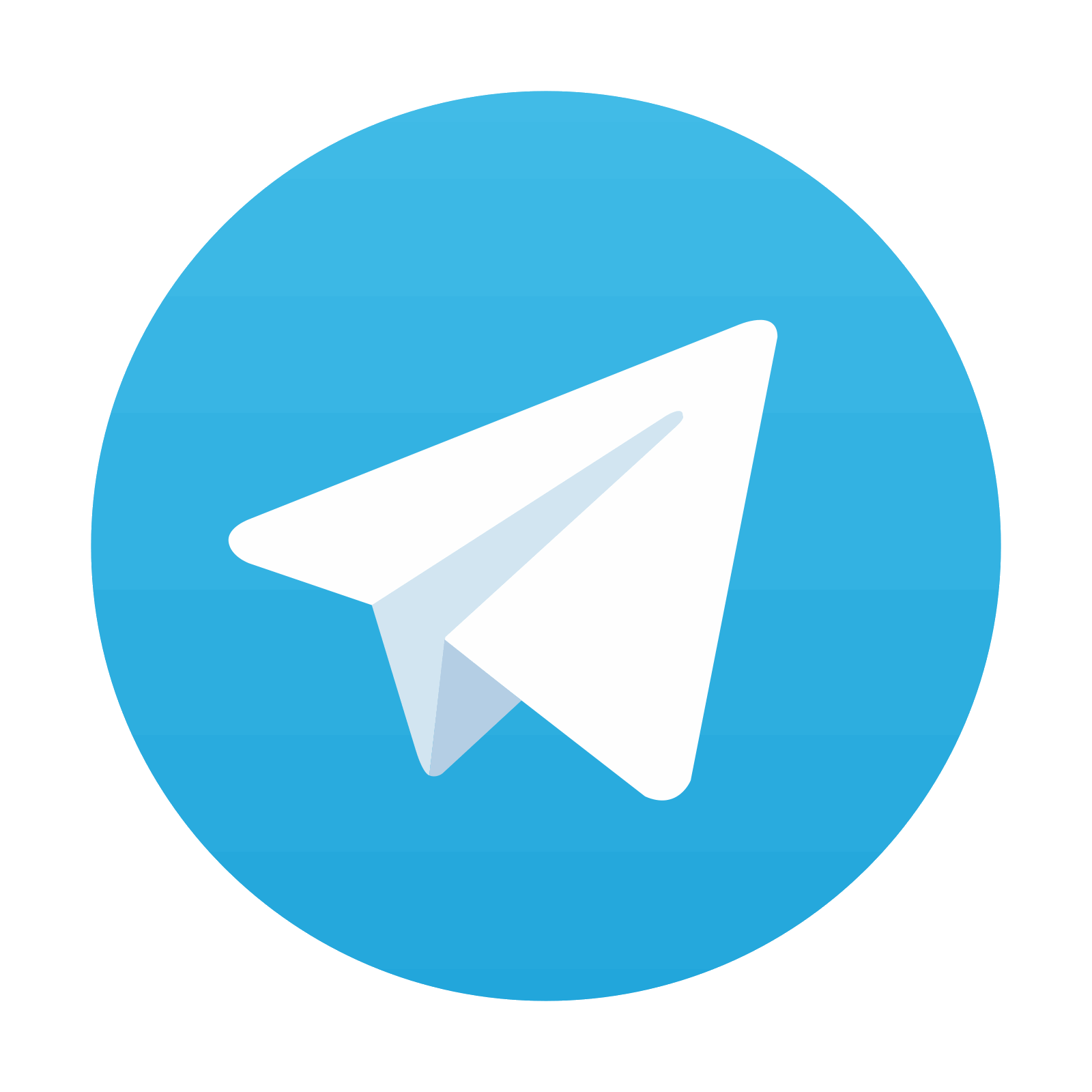
Stay updated, free articles. Join our Telegram channel
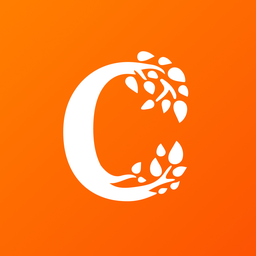
Full access? Get Clinical Tree
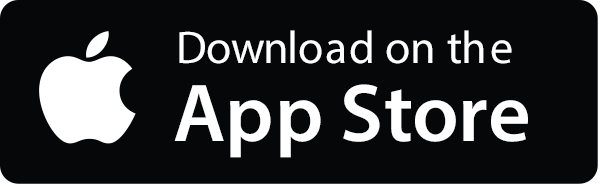
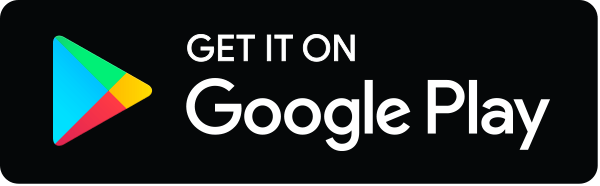
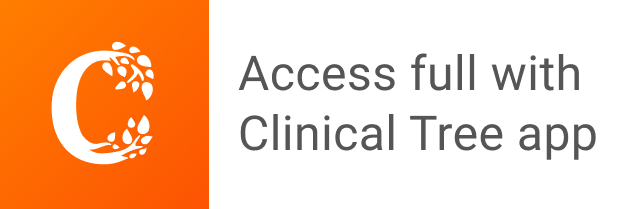