Abstract
Many new insights into the molecular mechanisms of intestinal absorption of calcium, phosphate, and magnesium have been made over the past decade. These include the identification, cloning, and characterization of transmembrane proteins responsible for the absorption of all three of these nutrient molecules. The proteins identified for uptake of Ca 2 + and Mg 2 + across the apical membrane of enterocytes are members of the transient receptor potential (TRP) family of cation channels (TRPV6 for Ca 2 + and TRPM6/7 heterotetramers for Mg 2 + ), while intestinal P i absorption across the apical membrane is now known to be mediated by a member of the sodium-phosphate cotransporter gene family (NaP i -IIb; SLC34A2 ). Additionally, in some cases, molecules responsible for movement of these molecules across the cellular cytoplasm (e.g., calbindins for Ca 2 + ) and exit across the basolateral membranes (e.g., plasma membrane calcium ATPase [PMCA1b] and the Na + /Mg 2 + exchanger) of intestinal epithelial cells have also been identified and extensively studied. With the discovery of these channels and transporters, we now have a much clearer picture of the molecular mechanisms involved in the transport of these crucial nutrient molecules. However, for all three of these molecules, transport proteins involved in transcellular uptake seem to be important only when intake levels are low, as at higher dietary intake levels, absorption occurs passively via still relatively obscure, paracellular transport pathways. Furthermore, additional information has shown that these transport processes are regulated by a number of physiological mediators, and that these effects are mediated by direct influences on the expression or activity of the identified transporters.
Keywords
Calcium, Phosphorus, Magnesium, Epithelium, Membrane transport, Na + -dependent transporter, Vitamin D, Channel, Diet, Nutrition
59.1
Introduction
Calcium, phosphorus, and magnesium belong to a group of essential chemical elements required to support a variety of biochemical processes by serving structural and functional roles. Although sophisticated homeostatic mechanisms have evolved to maintain serum levels, intracellular levels, and optimal mineral content in bone, disorders of mineral metabolism, including abnormalities of calcium, phosphorus, and magnesium homeostasis, are not uncommon. The homeostatic mechanisms affect primarily the three major target organs, the intestine, kidney, and bone. With parathyroid hormone (PTH) and vitamin D remaining the principal regulators, the emerging regulatory network including membrane transporters, associated proteins, and soluble mediators becomes increasingly more complex. In this chapter, we will focus primarily on the transport processes and proteins responsible for the absorption of all three of these nutrient molecules across the gastrointestinal tract. The transporters identified for uptake of calcium and magnesium across the apical membrane of enterocytes are members of the transient receptor potential (TRP) family of cation channels, while intestinal P i absorption across the apical membrane is now known to be mediated by a member of the sodium-phosphate cotransporter gene family ( SLC34 ). Additionally, molecules responsible for movement of these molecules across the cellular cytoplasm (e.g., calbindins for Ca 2 + ) and exit across the basolateral membranes (e.g., plasma membrane calcium ATPase [PMCA1b] for Ca 2 + ) of intestinal epithelial cells have also been identified and in some instances their functional relevance has been addressed in targeted knockout models. With the discovery of these channels and transporters, we now have a much clearer picture of the molecular mechanisms involved in the transport of these crucial nutrient molecules. The purpose of this chapter is thus to provide updated information regarding the molecular mechanisms of intestinal transport of calcium, phosphate, and magnesium.
59.2
Recommended Nutritional Requirements for Ca 2 + , Mg 2 + , and P i
In 1997, the Institute of Medicine (IOM) issued a comprehensive report that presented dietary reference values for intake of nutrients by Americans and Canadians ( Dietary reference intakes for calcium, phosphorus, magnesium, vitamin D, and fluoride. Standing Committee on the Scientific Evaluation of Dietary Reference Intakes, Food and Nutrition Board, Institute of Medicine. National Academy Press, Washington, DC, 1997; http://books.nap.edu/catalog/5776.html ). This report was part of a larger project that was conducted with the involvement of Health Canada, and other US governmental agencies that also supported the project. Since the publication of this report, there has been increasing interest in the possibility of enhanced roles for vitamin D and calcium in human health, thus resulting in increased calcium and vitamin D supplementation by the food industry and in the emerging
controversies concerning the adequate and safe levels of intake. In response to these trends, the Institute of Medicine conducted a review of data pertaining to calcium and vitamin D in an effort to identify Dietary Reference Intakes (DRIs) based on current scientific evidence regarding the roles of calcium and vitamin D in human health. At the time of the writing this chapter, a new report was available as a preprint pdf at http://www.nap.edu/ catalog.php?record_id=13050 ( Dietary reference intakes for calcium and vitamin D . Committee to Review Dietary Reference Intakes for Vitamin D and Calcium, Institute of Medicine of the National Academies, National Academy Press, Washington, DC, 2010).
DRIs are reference values that can be used to formulate and assess diets for healthy populations. The DRIs replace the periodic revisions of the Recommended Dietary Allowances (RDAs), which have been published since 1941 by the National Academy of Sciences. DRIs encompass the Estimated Average Requirement (EAR), the RDA, Adequate Intake (AI), and the tolerable Upper intake Level (UL). As has been the practice with dietary recommendations in the past from the Food and Nutrition Board (within the IOM), the DRIs that are included in these two reports apply to the general healthy population. RDAs and AIs are nutrient intake target levels that should decrease the risk of developing pathophysiological conditions related to that particular nutrient. Importantly, consideration of dietary practices associated with Ca 2 + and other nutrients has been limited to observations made within the United States and Canada, and may not be directly applicable to all populations.
The EAR is a nutrient intake value that is estimated to meet the requirement by 50% of the individuals in a particular life stage and gender group. At this intake level, the remaining 50% individuals would have either inadequate or excessive intake of a particular nutrient. In some cases, these recommendations have been extrapolated to estimate this value. The EAR is used in setting the RDA, and it may be one factor used for assessing and planning the adequacy of intake of various groups. The RDA is the average daily requirement that meets the nutritional needs of all individuals in a life stage and gender group (97%–98%) and is set two standard deviations above the EAR. It should be noted that the RDA applies to individuals, not groups. The EAR serves as the foundation for setting the RDA. Furthermore, the AI is set instead of an RDA if insufficient scientific evidence is available to calculate an EAR. The AI is based on observed or experimentally determined estimates of average nutrient intake by a group or groups of healthy people. The principle, intended use of the AI is as a goal for the nutrient intake of individuals. Additionally, the tolerable UL is the highest level of daily nutrient intake that will likely not pose any risk of adverse health effects to almost all individuals in the general population. This term is intended to set a level of intake that can, with high probability, be tolerated biologically. The UL is not intended to be a recommended level of intake.
The issue of calcium and vitamin D intake levels is still under debate. In the revised IOM report, the committee expanded the list of potential health indicators for both nutrients beyond positive calcium balance and optimal bone mineralization. Although their review took into consideration numerous emerging indications for calcium and vitamin D supplementation (i.e., cancer, cardiovascular diseases, diabetes, immunity, or neuropsychological functions), the conflicting nature of available evidence could not be used to establish health benefits with any level of confidence. Therefore, the evidence surrounding bone health continues to provide the most reasonable and supportable basis for DRI development. This is primarily relevant in the elderly, in whom intestinal calcium transport is declining, as are skeletal calcium reserves. In humans, intestinal absorption of calcium decreases with advancing age. Similarly, the physiological adaptation to low dietary calcium is also blunted in the elderly. These changes can be attributed to a progressive resistance to vitamin D that develops in the process of aging. In addition, renal production of 1,25(OH) 2 D 3 may also be impaired in the elderly. Consequently, the dietary intake of calcium required to maintain positive calcium balance progressively increases with advancing age.
In summary, the RDA or AI for an individual is the aim for the intake of a particular nutrient, while the UL should be used as a guide to limit intake. Chronic intake of amounts him excess of the UL may increase risk of adverse effects. The EAR is used to examine the possibility of inadequacy and to set the RDA. Evaluation of the true status of individuals however, requires clinical, biochemical, and/or anthropometric data. The findings of the two IOM reports and the current DRIs for dietary calcium, phosphorus, and magnesium for populations of various ages and genders are summarized in Table 59.1 .
Life Stage Group a | Calcium | Phosphorus | Magnesium | ||||||||||||
---|---|---|---|---|---|---|---|---|---|---|---|---|---|---|---|
EAR b | RDA c | UL d | AI e | EAR b | RDA c | AI e | EAR b | RDA c | AI e | ||||||
Male | Female | Male | Female | Male | Female | Male | Female | Male | Female | ||||||
0–6 months | – | – | – | – | 1000 | 210 | – | – | 100 | – | – | – | – | 30 | 30 |
7–12 months | – | – | – | – | 1500 | 270 | – | – | 275 | – | – | – | – | 75 | 75 |
1–3 years | 500 | 500 | 700 | 700 | 2500 | 500 | 380 | 460 | – | 65 | 65 | 80 | 80 | – | – |
4–8 years | 800 | 800 | 1000 | 1000 | 2500 | 800 | 405 | 500 | – | 110 | 110 | 130 | 130 | – | – |
9–13 years | 1100 | 1100 | 1300 | 1300 | 3000 | 1300 | 1055 | 1250 | – | 200 | 200 | 240 | 240 | – | – |
14–18 years | 1100 | 1100 | 1300 | 1300 | 3000 | 1300 | 1055 | 1250 | – | 340 | 300 | 410 | 360 | – | – |
19–30 years | 800 | 800 | 1000 | 1000 | 2500 | 1000 | 580 | 700 | – | 330 | 255 | 400 | 310 | – | – |
31–50 years | 800 | 800 | 1000 | 1000 | 2500 | 1000 | 580 | 700 | – | 350 | 265 | 420 | 320 | – | – |
51–70 years | 800 | 1000 | 1000 | 1200 | 2000 | 1200 | 580 | 700 | – | 350 | 265 | 420 | 320 | – | – |
> 70 years | 1000 | 1200 | 1200 | 1200 | 2000 | 1200 | 580 | 700 | – | 350 | 265 | 420 | 320 | – | – |
Pregnancy | |||||||||||||||
< 18 years | 1100 | 1300 | 3000 | 1300 | 1055 | 1250 | – | – | 335 | – | 400 | – | – | ||
19–30 years | 800 | 1000 | 2500 | 1000 | 580 | 700 | – | – | 290 | – | 350 | – | – | ||
31–50 years | 1000 | 580 | 700 | – | 300 | – | 360 | – | – | ||||||
Lactation | |||||||||||||||
< 18 years | 1100 | 1300 | 3000 | 1300 | 1055 | 1250 | – | – | 300 | – | 360 | – | – | ||
19–30 years | 800 | 1000 | 2500 | 1000 | 580 | 700 | – | – | 255 | – | 310 | – | – | ||
31–50 years | 1000 | 580 | 700 | – | – | 265 | – | 320 | – | – |
a All groups not specified are males and females, except pregnancy and lactation.
b EAR , Estimated Average Requirement (in mg/day). The intake that meets the estimated nutrient needs of 50% of the individuals in a group.
c RDA , Recommended Dietary Allowance (in mg/day). The intake that meets the nutrient needs of 97%–98% of individuals in a group.
d UL , Upper Intake Level (in mg/day). The highest level of daily nutrient intake that will likely not pose any risk of adverse health effects to almost all individuals in the general population.
e AI , Adequate Intake (in mg/day). For healthy infants fed human milk, AI is the estimated mean intake.
59.3
Intestinal Calcium transport
Calcium is recognized as a critical element for normal homeostasis and is a major constituent of bone. An average 70 kg adult human has 1 kg of body calcium, with more than 99% of calcium found in bones and teeth in the form of calcium hydroxyapatite (Ca 10 [PO 4 ] 6 [OH] 2 ). Bone tissue serves as a reservoir and a source of calcium for critical metabolic needs provided by Ca 2 + in the circulatory system, extracellular fluid, muscle, and other tissues where it is necessary for mediating vascular tension, muscle function, nerve transmission, intracellular signaling, and hormone secretion. All the calcium in the body is acquired from maternal and dietary sources; therefore, a thorough understanding of the molecular mechanisms responsible for intestinal calcium absorption is critical ( Fig. 59.1 ). Calcium deposition rates to the skeleton vary with age, with highest rates of deposition being found in the neonatal period, followed by minimal deposition once growth ceases. In general, bone calcium deposition parallels removal of calcium from bone, with the difference between the two processes constituting the bone calcium mass. Bone calcium mass is very low in the newborn period; it increases until 35–45 years of age, and then it gradually decreases with increasing age in men and it abruptly decreases in women several years after menopause. Calcium from the diet is absorbed throughout the small and large intestines, with an active transport mechanism being predominant in the proximal small intestine under conditions of low calcium intake. When calcium intake levels are high, calcium is absorbed along the length of the small and large intestines via a paracellular absorptive mechanism. Several excellent reviews have been published on this subject.

59.3.1
Calcium Supply and Bioavailability
Current estimates indicate that the median total calcium intake from all sources (food and dietary supplements) for persons > 1 year of age range from 918 to 1296 mg/day, depending upon life stage. Dairy products including milk, yogurt, and cheese are rich sources of calcium, providing the majority of calcium from foods in the general diet. In the United States, an estimated 72% of calcium comes from such dietary sources. The remaining calcium comes from vegetables (7%), grains (5%), legumes (4%), fruit (3%), meat, poultry, and fish (3%), eggs (2%), and miscellaneous foods (3%) (U.S. Department of Agriculture/Economic Research Service Nutrient Availability Data (2009); http://www.ers.usda.gov/Data/FoodConsumption/NutrientAvailIndex.htm ). Calcium supplementation of a number of foods that do not naturally contribute this mineral (e.g., orange juice, cereals) is becoming widespread and makes it difficult for the national food composition databases (such as those maintained by USDA) to remain current and likely leads to some underestimation of actual calcium intake from food sources. Moreover, about 43% of all people in the United States and almost 70% of older women reported calcium intake from supplements. When calcium supplement use is taken into account based on these survey data, the average intake increases by about 7% for males and 14% for females.
Controlled metabolic studies undertaken across a wide age range by the USDA and described by Hunt and Johnson determined that fractional calcium absorption (percentage of a given dose of calcium that is absorbed) in men and nonpregnant women is approximately 25% of calcium intake. The fractional calcium absorption is influenced by age, physiological state (e.g., pregnancy), and the level of Ca 2 + intake. It is also influenced by the chemical form of calcium and modulated by dietary constituents. Calcium must be in a soluble form in order to be absorbed by the gastrointestinal tract. Even in the alkaline conditions of the ileum where calcium salts may be formed, some calcium ions remain in solution. Total calcium absorption is dependent upon three factors: (1) local solubility, (2) the rate of transepithelial transport of calcium across the intestinal epithelium, and (3) the transit time of chyme movement through a particular gut segment. Furthermore, calcium in vegetables and other solid foods must be digested before going into solution and being available for transport. Thus, the calcium present in foods with high fiber content is likely to be poorly absorbed compared to a more easily digestible food source with identical calcium contents, although true digestibility is difficult to predict and actual calcium transport rates may be higher than expected.
The most common forms of supplemental calcium are calcium carbonate and calcium citrate. Calcium carbonate provides 40% of elemental calcium, compared with 21% for calcium citrate. This helps with compliance among the persons taking calcium carbonate supplements (fewer tablets needed and lower cost). However, compared with calcium citrate, calcium carbonate is more often associated with gastrointestinal side effects, such as constipation, flatulence, and bloating. Calcium citrate is also less dependent on low stomach pH for absorption and can be taken without food, by patients with achlorhydria, inflammatory bowel disease, or by patients with gastroesophageal reflux disease taking histamine-2 receptor blockers or proton-pump inhibitors. Several studies have reported on attempts to improve bioavailability of calcium by adding casein phosphopeptides to foods, or by the use of highly soluble salts such as calcium gluconate or calcium gluconate-glycerophosphate. Other studies have addressed the question of whether manipulation of certain diet components, such as adding rice cereal to infant formula or by increasing dietary fiber in weaning cereals, adversely affects calcium bioavailability or absorption. From many of these studies, it is now apparent that when calcium intake is adequate or high, differences in bioavailability, such as from increased solubilization, have little effect on intestinal calcium transport rates and calcium deposition to the skeletal system. However, under conditions of low dietary calcium intake and when calcium is present in a more insoluble form (such as in oxalate-rich spinach), the decrease in calcium transport becomes nutritionally significant. Several studies have sought to understand the high-absorbance rate of calcium from milk and suggested that lactose, phosphopeptides, and amino acids may play a role in this phenomenon. Interestingly, calcium bioavailability in some milk substitutes is decreased as compared to milk, despite the fact that nutritional quality is similar. Another area of interest is related to the effect of fat intake and the formation of calcium soaps on calcium bioavailability and intestinal transport rates. One study concluded that the addition of synthetic triglycerides to infant formula significantly improved calcium absorption in preterm neonates. However, the effect of fat intake on intestinal calcium homeostasis in older children and adults is not known. Furthermore, Sanyal et al. demonstrated that premicellar taurocholate, a component of bile, enhances calcium absorption from all regions of the rat small intestine.
A number of inorganic molecules and dietary components have also been recently reported to perturb intestinal calcium absorption. Phosphate may interfere with calcium absorption as a result of a formation of poorly soluble calcium-phosphate salts, but increasing dietary phosphate by a factor of 2.5 has no reported influence on calcium absorption, regardless of the amount of calcium consumed. However, indigestible organic phosphates, such as phytates of hexaphosphoinositol which are found in wheat bran, can form insoluble calcium salts. High concentrations of dietary magnesium can decrease calcium absorption presumably by competing for the calcium transport site, although we now know that calcium and magnesium are absorbed by different channel proteins. This effect could be due to interaction of magnesium ions with the specific calcium channels in the gut (TRPV6), as extracellular Mg 2 + has been shown to block the monovalent cation currents induced by TRPV6 in a heterologous expression system.
Certain antibiotics and cationic amino acids such as lysine stimulate intestinal calcium absorption. Similarly, lactose, other complex sugars, and glucose polymers promote calcium absorption. Although gastric acidity or achlorhydria was initially thought to have limited effects on intestinal calcium transport, there is an increasing concern that hypochlorhydria resulting from atrophic gastritis, bariatric surgery, or high-dose chronic use of proton pump inhibitors may create a risk for malabsorption of dietary and supplementary calcium and may increase the risk of osteopenia and osteoporosis in the long term.
Calcium is also known to be absorbed in the mammalian colon, with the cecum and the ascending colon having the highest rates; however, the transverse colon does not transport calcium. It has recently been demonstrated that acidic fermentation in the colon can enhance intestinal absorption of calcium and magnesium. Younes et al. concluded that the large intestine may represent a major site for calcium transport when acidic fermentation takes place. Likewise, another group demonstrated that fructooligosaccharides significantly enhanced calcium and magnesium absorption in the rat colon. These authors concluded that indigestible and fermentable carbohydrates facilitate colorectal absorption of these ions. Mineo et al. demonstrated that indigestible disaccharides directly affect the small intestinal and colonic epithelium by opening tight junctions between epithelial cells and thereby promoting Ca 2 + and Mg 2 + absorption. Additionally, another study showed that two fermentable carbohydrates (inulin and resistant starch) showed synergistic effects on intestinal calcium absorption and calcium balance in rats. Calcium absorption may also be enhanced by acetate and propionate in the human rectum and distal colon, by a nonsaturable, diffusive process.
The net epithelial transfer of calcium represents the sum of two opposing processes: transepithelial absorption and the loss of calcium in shed mucosal cells and in secretory fluids (saliva, gastric and pancreatic juices, and bile). Thus, fecal calcium contains unabsorbed calcium from dietary sources as well as endogenously excreted calcium. Abrams et al. estimated the endogenous fecal calcium losses at approximately 2.1 mg/kg/day in adults and 1.4 mg/kg/day in children. In contrast to urinary excretion, endogenous fecal Ca 2 + excretion is not significantly influenced by aging.
59.3.2
Molecular Mechanisms of Intestinal Calcium Transport
Depending on the segment and the luminal Ca 2 + concentration, absorption occurs via a weakly regulated paracellular route, or by a highly regulated and 1,25(OH) 2 vitamin D 3 -sensitive transcellular pathway. When dietary Ca 2 + intake and luminal concentration is low (< 20 mM), active transcellular transport in the duodenum predominates and accounts for approx. 80% of the total Ca 2 + absorption. At higher Ca 2 + supply (> 50 mM), the contribution of active transport diminishes to > 10%, largely due to the short duodenal transit time and downregulation of the key molecular components of the transcellular Ca 2 + transport pathway. In addition to calcium content in the diet, the amount of Ca 2 + transferred paracellularly is directly related to its solubility (decreasing in the distal small intestine), the sojourn of the chyme in a given intestinal segment (highest in the ileum), and the rate of paracellular diffusion from lumen to blood. Age is also an important factor determining the primary Ca 2 + absorption route. In newborns, it is largely passive and facilitated by the lactose content of breast milk. Ghishan et al. and Halloran and DeLuca demonstrated that as the neonate grows, passive absorption declines and calcitriol-stimulated active intestinal calcium absorption becomes more important. Three complementary models of transcellular Ca 2 + absorption have been proposed: a facilitated diffusion model, a vesicular transport model, and tunneling through the endoplasmic reticulum. All four modes of intestinal Ca 2 + transport are systematically discussed below.
59.3.2.1
The Paracellular Pathway of Dietary Calcium Absorption
Although paracellular Ca 2 + transport is the major route of absorption in the intestine with high-calcium diets, its importance or regulation has not been investigated in great detail. It is generally believed that contrary to the transcellular pathways, paracellular Ca 2 + transport is not tightly regulated. However, recent years have brought an increasing appreciation of the complexity and plasticity and dynamics of tight junction (TJ) assembly, and of the role of TJ components in paracellular Ca 2 + permeability. Biochemical and physiological aspects of tight junctions are reviewed in more detail elsewhere in this book (T. Ma “Gut Barrier: Tight Junctions”). Kutuzova and Deluca identified a number of 1,25-(OH) 2 D 3 target genes whose protein products are localized in the vicinity of intestinal tight junctions and which could influence TJ integrity and permeability. These included not only transporters and channels but also several intra- and intercellular matrix-related proteins and G-proteins. There were also a number of 1,25-(OH) 2 D 3 downregulated genes encoding proteins that are involved in the regulation of tight junction permeability, including sodium-potassium ATPase, claudin 3, aquaporin 8, cadherin-17, and RhoA. The conclusion was drawn that 1,25-(OH) 2 D 3 may increase intestinal epithelial tight junction permeability or modulate their selectivity toward Ca 2 + and other cations by regulating expression of proteins involved in tight junction formation. The increased tight junction permeability and/or selectivity, regulated by 1,25-(OH) 2 D 3 , could direct Ca 2 + absorption through the tight junction-regulated paracellular pathway in the intestinal epithelium. This suggestion is in agreement with a recent study that demonstrated that 1,25-(OH) 2 D 3 stimulated an increase in tight junction conductance, and increased paracellular Ca 2 + , Na + , Rb + , and mannitol transport in an enterocyte-like cell line (Caco-2). Under these conditions, no significant contribution of the Ca 2 + -ATPase-mediated transcellular pathway to overall transepithelial Ca 2 + transport was detected. More recently, Fujita et al. showed that 1,25-(OH) 2 D 3 upregulates claudin-2 and claudin-12 in intestinal epithelial cells via a VDR-dependent mechanism and that both claudins contribute to the vitamin D-induced increase in paracellular Ca 2 + permeability. Claudin-16 (paracellin 1) has also been implicated in playing a significant role in paracellular permeability of Ca 2 + and Mg 2 +, and mutations within the claudin-16 gene have been linked with familial hypomagnesaemia with hypercalciurea and nephrocalcinosis (FHHNC). However, due to a limited pattern of expression (selective expression at tight junctions of renal epithelial cells of the thick ascending limb of the Henle loop), the role of claudin-16 in intestinal Ca 2 + permeability is unlikely.
Additional evidence has accumulated demonstrating that 1,25-(OH) 2 D 3 enhanced both cell-mediated active and passive, paracellular Ca 2 + and P i transport in a Ussing chamber study with rat small intestine and for Ca 2 + transport in Caco-2 cell monolayers. More recently, Tudpor et al. demonstrated nontranscriptionally mediated, short-term increase in paracellular Ca 2 + transport across rat duodenum treated in vitro with 1,25-(OH) 2 D 3 . This solvent drag-induced increase in Ca 2 + transport was dependent on phosphatidylinositol 3-kinase (PI3K), protein kinase C (PKC), and mitogen-activated protein (MAP) or extracellular signal-regulated (Erk) kinase (MEK) activity. Unlike the passive, Ca 2 + gradient-directed transport, solvent drag-induced paracellular Ca 2 + absorption resembles a secondary active transport in that it is dependent on the activity of Na + /K + -ATPase that creates a paracellular hyperosmotic gradient resulting from a sodium concentration ~ 15 mM higher than the surrounding milieu and is stimulated by high glucose level.
59.3.2.2
Facilitated Diffusion: Apical Ca 2 + Entry
Early studies indicated that over a wide range of transepithelial Ca 2 + transport rates, Ca 2 + influx at the apical membrane is correlated in a 1:1 fashion with the apical to basolateral Ca 2 + flux. Ca 2 + entry was postulated to occur via Ca 2 + -selective channels at the luminal membrane, under the influence of a steep inwardly directed electrochemical gradient. Initial studies demonstrated that 1,25(OH) 2 D 3 affected Ca 2 + influx in enterocyte-like cells. This calciotropic hormone significantly increased radiolabled Ca 2 + uptake within several minutes in a dose-dependent manner in rat, intestinal epithelial cells. These and other studies suggested that a Ca 2 + channel was activated by 1,25(OH) 2 D 3 through a cAMP/PKA-dependent pathway in the mammalian intestine.
Further studies utilized functional expression cloning in an attempt to identify the apical calcium influx transporter/channel. The first such experiments were done by Hoenderop et al., who utilized a cDNA library constructed from poly(A) + RNA isolated from primary cultures of rabbit kidney cells. The cDNA clones were copied into cRNA, which was microinjected into Xenopus laevis oocytes, followed by screening for Ca 2 + uptake activity in the presence of a cocktail of known voltage-gated Ca 2 + channel inhibitors (the renal and intestinal Ca 2 + channels were known to NOT be voltage-gated). After extensive screening procedures, a single transcript was isolated which encoded a novel epithelial Ca 2 + channel (first called ECaC1 and recently renamed as transient receptor potential channel TRPV5; also known as ECaC and CaT2). When the TRPV5 channel was expressed in Xenopus oocytes and in HEK293 cells (human, embryonic kidney cells), calcium transport was inhibited by (in order of potency) La 3 + > Cd 2 + > Mn 2 + , whereas Ba 2 + and Sr 2 + had no effect. Permeability to Na + was negligible in the presence of Ca 2 + . Moreover, the TRPV5 channel was shown to be induced by 1,25(OH) 2 D 3. Overall, these observations demonstrated that TRPV5 possesses all the expected characteristics of the calcium influx in Ca 2 + -transporting epithelia. The TRPV5 transporter was subsequently determined to be expressed predominantly in the kidney and was conclusively shown to be responsible for renal Ca 2 + reabsorption.
Subsequent studies by Hediger and coworkers applied the functional expression cloning technique to the search for the intestinal calcium entry channel, and they were able to identify an intestinal Ca 2 + transporter, which they called CaT1. CaT1 shares 80% amino acid sequence identity to TRPV5. CaT1 has subsequently been renamed TRPV6 and is also known in the literature as ECaC2 and CaT-like. Electrophysiological studies, as described in detail below, demonstrated that the characteristics of TRPV6 are comparable to those measured for TRPV5, but the expression pattern of TRPV6 is more ubiquitous. Moreover, functional properties of both TRPV5 and TRPV6 are in congruence with the known properties of the putative epithelial calcium channels, which are responsible for intestinal and renal calcium (re)absorption. TRPV6 is currently thought to be the major player in active, intestinal calcium transport.
59.3.2.2.1
The Transient Receptor Potential (TRP) Family of Channel Proteins
TRP channel proteins form a large and diverse, but related family of proteins that are expressed in many tissues and cells types. The large functional diversity of this family of proteins is reflected in their variable permeability to ions, activation mechanisms, and their involvement in a wide range of physiological processes. The TRP channels can be divided by sequence homology into at least six subfamilies, designated TRPC (canonical or classical), TRPV (vanilloid), TRPM (melastatin), TRPP (polycystins, PKD-type), TRPA (for ankyrin), TRPML (for mucolipin), and the more distantly related subfamily TRPN (N for “nomp,” no mechanoreceptor potential). An intriguing subfamily within the TRP superfamily is the TRPV family, consisting of six members. This group of channels includes TRPV1-4, which respond to heat, osmolarity, odorants, and mechanical stimuli, whereas TRPV5 and 6 are epithelium Ca 2 + channels and have been implicated in maintaining body-Ca 2 + balance by facilitating Ca 2 + (re)absorption in the kidney and small intestine.
Extensive scientific evidence supports the concept of paracellular transport of calcium with high dietary intake levels, as described earlier in this chapter. However, Morgan et al. reported the discovery of another intestinal calcium channel (Ca v 1.3; CACNA1D), homologous to the neuroendocrine L-type Ca v 1.3 calcium channel. This group’s immunocytochemical studies demonstrated its expression on the apical membrane of enterocytes from the proximal jejunum to the mid-ileum, and our own observations further determined Ca v 1.3 expression in the human and mouse colon. Perfusion studies with 1.25 mM luminal calcium revealed L-type calcium channel activity; this activity was inhibited by phloridizin, nifedipine and Mg 2 + , and activated by Bay K 8644. These findings demonstrated channel-mediated rather than paracellular calcium transport. Furthermore, none of these compounds affected the expression of the active calcium transport channels (e.g., TRPV5 and TRPV6; discussed below). These authors thus conclude that intestinal Ca v 1.3 may mediate a significant route of calcium transport during times of dietary calcium sufficiency. In contrast to other channels such as TRPV6 that work under a hyperpolarized setting, Ca v 1.3 is hypothesized to contribute to an alternative, TRPV6-independent, route of intestinal Ca 2 + absorption. While TRPV6 plays a more dominant role under the polarizing conditions between meals, Ca v 1.3 plays a dominant role under postprandial depolarizing conditions, such as during digestion, when nutrients and luminal Ca 2 + are abundant. In in vitro studies with Caco-2 cells, Nakkrasae et al. showed that Ca v 1.3 was the sole apical channel responsible for the prolactin-stimulated transcellular calcium transport. The recently reported phenotype of Ca v 1.3-null-knockout mice includes a smaller skeleton, lower body weight, and lower bone mineral content. However, intestinal Ca 2 + absorption has not been measured in these mice and since Ca v 1.3 is expressed in multiple tissues, including osteoblasts, the role of this Ca 2 + channel in intestinal epithelial cells would have to be more precisely elucidated in a tissue-specific knockout model, especially that the contribution of Ca v 1.3 to either basal or vitamin D-regulated intestinal Ca 2 + absorption in vivo has been recently questioned by Reyez-Fernandez and Fleet.
59.3.2.2.2
TRPV5 and TRPV6
The epithelial Ca 2 + channel family is restricted to two members, and genomic cloning demonstrated that TRPV5 and TRPV6 channels are transcribed from distinct genes. Interestingly, TRPV5 and 6 are juxtaposed on human chromosome 7q35, with a distance of only 22 kb separating them, which suggests that a single ancestral gene was duplicated over evolutionary time. An analogous situation was observed in the mouse genome, in which the two genes are located close to one another on chromosome 6, in a region that is syntemic to human chromosome 7q33-35. To date, TRPV5 and TRPV6 have been cloned from many species, including rabbit, rat, mouse, and human. The putative proteins exhibit an overall amino acid sequence homology of 75%–80%. Interestingly, several domains within these proteins are completely conserved between species, including the membrane topology of the protein with six putative transmembrane segments and the postulated pore region. Furthermore, detailed sequence analysis of these proteins identified several putative phosphorylation sites, for PKC-, PKA-, and cGMP-dependent kinase. Phosphorylation of Thr 709 by cAMP-dependent protein kinase A has recently been reported to mediate PTH-induced channel’s opening probability and Ca 2 + reabsorption in the kidney. Another example of phosphorylation-dependent regulation of TRPV5 was provided by Topala et al., who showed that in renal epithelial cells, activation of the extracellular calcium sensing receptor (CaSR) stimulates TRPV5-mediated Ca 2 + influx via a PMA-insensitive PKC-mediated phosphorylation of Ser 299 and Ser 654 . While TRPV6 was not affected by CaSR activation, phosphorylation may also play a role in regulating this primarily intestinal Ca 2 + channel. Inhibition of tyrosine phosphatases increases TRPV6 activity—an effect abolished by mutations Tyr 161 and Tyr 162 . Moreover, ATP stabilizes TRPV6 Ca 2 + current, a phenomenon that can be reversed by PKC stimulation with phorbol ester (PMA). Two residues at both the N- and C-termini, Ser 144 and Thr 688 , synergistically contribute to mediate the effect of PMA; these two amino acids have been postulated to form ATP binding domains, allowing to form a bridge between the termini and modulating TRPV6 channel properties.
Additionally, both the TRPV5 and six proteins contain PDZ motifs and ankyrin repeats in the NH 2 -terminal region, which are conserved in a diverse range of receptors and ion channels, including the TRP superfamily. PDZ motifs are recognized by proteins containing PDZ interacting domains, and these protein-protein interactions may be involved in protein targeting and multiprotein complex assembly. PDZ domain interactions serve not only scaffolding and cytoskeletal attachment roles but there is also evidence that they can regulate the functions of their ligands. PDZ domain-containing proteins typically interact with COOH-terminal PDZ motifs in target proteins, but interactions could also occur with the N-terminal motifs that are present in TRPV5 and 6. Ankyrin repeats have similar roles as PDZ domain/motif interactions, in that they can link transporters and cell adhesion molecules to spectrin-based cytoskeletal elements in specialized membrane domains. Kim et al. proposed that PDZK2 is an essential TRPV6-interacting protein and a physiological modulator of TRPV6 activity. A direct interaction between the TRPV6 C-terminal PDZ-binding motif and PDZK2 was identified by GST pull-down assay. Although heterologous overexpression of both TRPV6 and PDZK2 did not affect the activation of TRPV6, mutation of the four critical residues in TRPV6 (EYQI) decreased peak current amplitude of the channel and RNAi-mediated knockdown of endogenous PDZK2 in HEK293 cells significantly decreased current density in divalent-free media (DVF).
Several studies addressed the expression of TRPV5 and TRPV6 in the gastrointestinal tract. Initially, Northern blot analysis showed expression of rabbit TRPV5 in duodenum and jejunum, whereas ileum was negative. However, these hybridizations were performed prior to the identification of the TRPV6 isoform, using full-length cDNA probes that did not discriminate between the highly homologous TRPV5 and TRPV6 transcripts. Subsequent experimental approaches using isoform-specific probes, quantitative PCR analysis, and immunohistochemical studies found expression of both channels in the intestine. However, these studies demonstrated that TRPV6 transcript levels in the gut are at least three orders of magnitude higher than TRPV5 transcript levels. Another study addressed the issue of whether TRPV5 has an important physiological role in the intestine, by creating TRPV5-knockout mice. These animals exhibit intestinal Ca 2 + hyperabsorption, most likely mediated by increased TRPV6 and calbindin-D 9k expression levels, which suggests a predominant role for TRPV6 in intestinal calcium transport. Consistent with this observation, targeted deletion of the TRPV6 gene resulted in perturbed Ca 2 + homeostasis, including a 60% decrease in intestinal Ca 2 + absorption, deficient body weight gain, decreased bone mineral density, and reduced fertility. Changes in bone density were later associated with increased bone resorption driven by increased osteoclasts differentiation and activity in TRPV6 −/− mice. Similarly, homozygous knock-in of a D541A mutation in the TRPV6 pore region into mouse TRPV6 gene resulted in significantly impaired intestinal Ca 2 + uptake despite an increase in duodenal TRPV5 expression during feeding with low Ca 2 + diet.
In the mammalian intestine, TRPV6 expression is found in the duodenum, jejunum, cecum, and colon where it is colocalized in epithelial cells along with other molecular components of intestinal calcium absorption, calbindin D 9k , and PMCA1b. One study conducted by Hediger and coworkers demonstrated expression of TRPV6 throughout the entire digestive tract from esophagus to colon. Additional studies estimated TRPV6 and TRPV5 mRNA expression levels in the mouse by quantitative PCR analysis, and resulting data were normalized for the amount cDNA used for the amplification. This study demonstrated that TRPV6 mRNA expression was highest in duodenum and cecum, lower in the colon, and even lower in the ileum. This investigation also demonstrated that TRPV5 mRNA was expressed at much higher levels in the kidney as compared to the duodenum and cecum, whereas ileum and colon did not express TRPV5 mRNA. Immunohistochemical techniques have also been used to determine the distribution of TRPV6, calbindin D 9k , and PMCA1b proteins in the small intestinal epithelium. TRPV6 was localized along the brush-border membrane, whereas calbindin D 9k was found in the cellular cytoplasm and PMCA1b was expressed at the basolateral membrane. Further detailed immunolocalization studies demonstrated expression of TRPV6 on the apical membrane of enterocytes in the entire small intestine and colon.
When considered in their entirety, the current data strongly suggest that the epithelial Ca 2 + channel TRPV6 is the major transcellular mediator of Ca 2 + uptake from the intestinal lumen. Thus, the remainder of this section will focus on this intestinal calcium channel.
59.3.2.2.3
TRPV6 Molecular Structure and Protein-Protein Interactions
Crystal structure of TRPV6 has been described by Saotome et al., and the structural elements and requirements for activity of TRPV5 and TRPV6 channels have been recently reviewed by van Goor et al. TRPV6 likely forms homotetramers in the plasma membrane of cells. This tetrameric organization closely resembles the structure of the Shaker potassium channel, which is composed of four tandemly associated homologous domains. The clustering of the four subunits is thought to create an aqueous pore centered at the fourfold symmetry axis. This proposed tetrameric architecture implies that aspartic acid residues D542 and D541 form a negatively charged ring structure that functions as a calcium-selectivity filter, analogous to voltage-gated calcium channels. Recently, Niemeyer et al. identified the third ankyrin repeat in TRPV6 as being critical for physical assembly of TRPV6 subunits into the tetrameric form. Deletion or mutation of amino acid residues within this ankyrin repeat renders the channel nonfunctional and abolishes tetrameric formation. It was suggested that the third ankyrin repeat initiates a molecular zippering process that proceeds past the fifth ankyrin repeat and creates an intracellular anchor that is necessary for assembly of the functional subunits. However, in a recent report by Phelps et al., which provided detailed mapping and crystal structure of the N-terminal ankyrin repeat domain (ARD) of TRPV6, TRPV6-ARD’s were monomeric in solution. Furthermore, the packing and symmetry of the TRPV6-ARD crystals seemed incompatible with tetrameric assembly of the ARD around a fourfold symmetry axis. While previous studies demonstrated that the integrity of the ARD is important in channel assembly, this study indicates that it is not mediated through self-tetramerization of the ARD. The authors hypothesized that TRPV6 assembly may be assisted by additional cellular factors, which require the ARD but are unable to bind an ARD destabilized by mutation or partial deletion.
In addition to forming homotetramers, TRPV5 and TRPV6 can form heterotetramers. This observation is based upon cross-linking studies, coimmunoprecipitations and molecular mass determination of TRPV5/6 complexes using sucrose gradient sedimentation. When these two isoforms are coexpressed in some tissues, they may oligomerize, and this hetero-oligomerization may influence the functional properties of the Ca 2 + channels formed. As both these proteins exhibit different channel kinetics with respect to Ca 2 + -dependent inactivation, Ba 2 + selectivity and sensitivity for inhibition by ruthenium red, the influence of the heterotetrameric composition on channel properties could be important in certain tissues and cell types. In the intestine, however, TRPV6 has been shown to be expressed at levels as much as 100–1000 times higher than TRPV5, and no experimental evidence has directly demonstrated that there is actually heterotetramerization of the two channels in the intestine.
A number of regulatory proteins have been described that modify the activity, and biophysical and pharmacological properties of ion channels and transporters by direct, physical interactions, including TRPV5 and TRPV6. An auxiliary protein of TRPV6 was identified by screening a mouse kidney cDNA library using the yeast two hybrid system . This study identified S100A10 as a protein that specifically associates with the carboxy-terminus of the TRPV6. S100A10 is a 97 amino acid protein, which is a member of the S100 superfamily, that is present in vertebrates, insects, nematodes, and plants. This protein is predominately present as a heterotetrameric complex with annexin 2, which has been implicated in numerous biological processes including endocytosis, exocytosis, and membrane-cytoskeletal interactions. A recent report suggested a regulatory role for the S100A10-annexin 2 heterotetramer in vitamin D-mediated, intestinal calcium transport and in TRPV6 function and/or regulation. The association of S100A10 with TRPV6 was restricted to a short-peptide sequence NH 2 -VATTV-COOH located in the carboxy-terminus of the channel, a region that is conserved across species. Intriguingly, the NH 2 -TTV-COOH sequence in the putative S100A10 binding motif of TRPV6 resembles an internal, type I PDZ consensus binding sequence (NH 2 -S/TXV-COOH). However, S100A10 does not contain PDZ domains, suggesting that the interaction with TRPV6 is distinct. The first threonine of the S100A10 interaction motif is crucial for binding to TRPV6. In fact, the activity of TRPV6 was abolished when this particular residue was mutated, demonstrating the necessity for calcium channel function. Furthermore, these mutant channels were mislocalized within cells, indicating that the S100A10-annexin 2 heterotetramer facilitates the translocation of TRPV6 channels to the plasma membrane. The importance of annexin 2 in the process was demonstrated by siRNA-mediated knockdown of annexin 2, which significantly inhibited the currents through TRPV6, exemplifying that annexin 2 in conjunction with S100A10 is necessary for normal TRPV6 activity. More recently, Borthwick et al. found that formation of the annexin 2-S100A10-TRPV6 complex was dependent on forskolin-induced and calcineurin (CnA)-dependent dephosphorylation of annexin 2 in lung and intestinal epithelial cells. This study demonstrated that cAMP/PKA/CnA signaling was important for annexin 2-S100A10 complex formation and interaction with target molecules in both absorptive and secretory epithelia.
Interestingly, similar to the epithelial calcium channels, S100A10 expression was found to be vitamin D sensitive. In addition, annexin 2 expression levels have been shown to increase with 1,25(OH) 2 D 3 treatment. Thus, physical interaction and coregulation of TRPV6 with S100A10 and annexin 2 could control trafficking of these channels to the plasma membrane. 1,25(OH) 2 D 3 has been shown to act via rapid nongenomic and slower genomic actions. The genomic effects are mediated by interaction with nuclear VDR/RXR heterodimers. Recently, it was reported that annexin 2 serves as a membrane receptor for 1,25(OH) 2 D 3 and that it mediates a rapid effect of the hormone on intracellular calcium homeostasis. It was shown that that 1,25(OH) 2 D 3 specifically bound to annexin 2 on the plasma membrane of rat osteoblast-like cells. Partially purified plasma membrane proteins and purified annexin 2 exhibited specific, saturable binding for tritiated 1,25(OH) 2 D 3 . These results suggest that annexin 2 may serve as a receptor for rapid actions of 1,25(OH) 2 D 3 ; however, this concept is still under debate. Taken together, these findings show that the S100A10-annexin 2 complex is required for the trafficking of TRPV6 to the plasma membrane and therefore is involved in overall calcium homeostasis. In addition to association with annexin 2, TRPV6 has also been found to associate with cyclophilin B (CypB) in the human placenta. When coexpressed in Xenopus oocytes, CypB increased TRPV6-mediated calcium uptake, a phenomenon that could be inhibited with CypB inhibitor, cyclosporin A. Although, as with most cyclophilins, CypB is ubiquitously distributed, its role in intestinal Ca 2 + transport has not been elucidated.
59.3.2.2.4
Physiology of TRPV6-Mediated Ca 2 + Transport
In the intestinal lumen, Ca 2 + concentration varies, but it is often in the millimolar range, while inside the absorptive cell, the Ca 2 + concentration is much lower (~ 100 nmol/L). This gives an approximate 10,000-fold concentration gradient across the apical membrane, and moreover, the membrane electric potential provides an additional driving force for calcium transport (with a relative negative charge on the cytoplasmic side of the membrane). Thus, transport of Ca 2 + into intestinal epithelial cells does not require the consumption of metabolic energy. Ca 2 + influx mediated by TRPV6 does not appear to be coupled with NaCl or protons. Transport activity is sensitive to pH, with Ca 2 + uptake activity increasing at alkaline pH. TRPV6 is permeable to Ba 2 + and Sr 2 + , but not Mg 2 + . Its apparent affinity for Ca 2 + ( K m ) is 0.44 mM.
The macroscopic properties of this channel expressed in X. laevis oocytes indicate that this protein works as a facilitative uniporter, that constitutively transports substrate down the concentration gradient with saturation kinetics, but without obvious gating mechanisms. Basic electrophysiological studies for TRPV6 have shown that outward currents are extremely small, indicating the channel is nearly completely inwardly rectifying. The current through TRPV6 is carried exclusively by Ca 2 + at extracellular Ca 2 + concentrations exceeding 10 μM. TRPV6 shows high selectivity for calcium, with Ca 2 + to Na + permeability ratios ( P Ca 2 + / P Na + ) of over 100. This channel is effectively blocked by trivalent and divalent cations, and is relatively insensitive to L-type voltage gated channel blockers (as discussed in detail below).
59.3.2.2.5
Pharmacology of TRPV6
Relatively little is known about effective pharmacological tools to modulate TRPV6 activity. Examination of Ca 2 + channel blockers revealed sensitivity toward ruthenium red, Gd 3 + , and La 3 + . The inhibition magnitude increased as follows: Gd 3 + , La 3 + > Pb 2 + , Cd 2 + > Co 2 + , Ni 2 + . These findings are consistent with studies on Ca 2 + current and flux in the small intestinal epithelium. Furthermore, TRPV6 is rather insensitive to the L-type voltage-gated calcium channel blockers nifedipine, diltiazem, and verapamil, with TRPV6 being inhibited only 10%–15% when these compounds were used at 100 μM. Econazole, miconazole, and SKF96365 were shown to inhibit Ca 2 + uptake in TRPV6 expressing oocytes, with econazole being the most effective inhibitor (~ 50% inhibition with 50 μM). The inorganic polycationic dye ruthenium red, which binds to phospholipids, inhibits TRPV6 in a voltage-dependent manner. Furthermore, xestospongin, a noncompetitive inositol 1,4,5-triphosphate receptor antagonist, seems to block TRPV6 activity as well. Additionally, capsaicin has been reported to block TRPV6. 2-APB (2-aminoethoxydiphenyl borate), a store-operated calcium channel (SOC) inhibitor also effectively inhibited human TRPV6 but not TRPV5. Searching for more specific inhibitors, Simonin et al. described cis -22a through an in silico method of ligand-based virtual screening. No direct activator of TRPV5 or TRPV6 has been identified to date. The pharmacology of TRP cation channels, including TRPV5 and TRPV5, was reviewed in detail by Vriens et al.
59.3.2.3
Facilitated Diffusion: Calcium Diffusion Across the Intestinal Epithelial Cell Cytoplasm
Epithelial cells involved in transcellular calcium transport are continuously challenged by substantial Ca 2 + moving through the cytosol, while the cells simultaneously need to maintain very low levels of intracellular Ca 2 + . Free ionized cytoplasmic Ca 2 + concentrations have to be tightly controlled due to cytotoxic effects, and due to potential inhibitory effects on TRPV5 and TRPV6-mediated Ca 2 + currents. Some of these effects are mediated by associated proteins like calmodulin and the protein kinase C substrate 80K-H. The facilitated Ca 2 + diffusion model proposes binding of calcium to an intracellular buffering protein, which delivers the calcium to the basolateral membrane for export. Mathematical modeling predicts that intracellular diffusion of this protein/Ca 2 + complex is the rate-limiting step in transepithelial calcium movement. Indeed, the 1,25(OH) 2 D 3 -dependent Ca 2 + -binding protein calbindin-D 9k (CBD 9k ) has been detected in intestinal epithelial cells and has been proposed to deliver calcium to the basolateral membrane for export into the interstitial space. The expression level of calbindin-D 9k in the intestinal epithelium closely correlates with the efficiency of Ca 2 + absorption, and therefore, this protein plays a central role in the facilitated diffusion model. Interestingly, calbindin D 9k may directly enhance plasma membrane Ca 2 + -ATPase (PMCA) activity, which is the principle mechanism of calcium export across the basolateral membrane.
Calbindin-D 9k belongs to a group of intracellular proteins that bind Ca 2 + with high affinity, which causes the protein to undergo structural changes due to electrostatic interactions. Another similar protein called calmodulin (CaM) is known to interact with and regulate the activity of voltage-gated Ca 2 + channels in a Ca 2 + -dependent fashion. The ubiquitously expressed CaM directly interacts with an “IQ motif” present in the carboxy-terminal of these channels, where it functions as a Ca 2 + sensor. This IQ motif, however, is not present in TRPV6. It is unknown whether calbindin-D 9k can perform a similar Ca 2 + sensor function for which a specific interaction with TRPV6 would be required. Furthermore, the striking colocalization of calbindin-D 9k with TRPV6 in the intestine suggests that a functional interaction between these two proteins may occur. Further experiments are needed to delineate whether the function of calbindin-D 9k is restricted to its buffering capacity to maintain low Ca 2 + concentrations in the cell in close vicinity to the channel opening, or whether physical interaction between calbindin-D 9k and TRPV6 is needed to exert a direct regulatory function.
Despite the large body of literature on the role of calbindins D 9k acting both as a Ca 2 + buffer and a ferry facilitating trans-cytoplasmic movement of Ca 2 + to the basolateral site in the intestinal epithelial cells, its precise role in both functions remains controversial. Interestingly, TRPV6 −/− , CBD 9k −/− , or TRPV6 −/− /CBD 9k −/− double-knockout mice had normal plasma Ca 2 + . In the same report by Benn et al., under low dietary calcium conditions, wild type, TRPV6 −/− and CBD 9k −/− mice showed a 4.1-, 2.9-, and 3.9-fold increase in duodenal calcium transport, respectively. A significant, 2.1-fold increase in duodenal calcium transport was also observed in TRPV6 −/− /CBD 9k −/− double-knockout mice fed a low-calcium diet. 1,25(OH) 2 D 3 administration to vitamin D-deficient null mutant and wild-type mice also resulted in a significant increase in duodenal calcium transport. These studies with genetically engineered mice challenge the dogma that TRPV6 and calbindin-D 9k are essential for vitamin D 3 -induced active intestinal calcium transport and imply that either naturally or through compensatory mechanisms, active intestinal Ca 2 + transport can occur in the absence of TRPV6, CBD 9k or both. Alternative transport models described below (vesicular transport model and Ca 2 + tunneling through ER) may partially explain the lack of a significant phenotype in CBD 9k -deficient mice.
59.3.2.4
Facilitated Diffusion: Calcium Extrusion Across the Basolateral Membrane
Two transport proteins have been implicated in the cellular exit of Ca 2 + . One of them is a bi-directional Na + /Ca 2 + exchanger NCX1, which under physiological electrochemical gradients, is responsible for basolateral Ca 2 + extrusion. It is widely expressed in absorptive epithelia, although it is considered to be of primary importance in the renal distal convoluted tubules, and of minor significance in the intestinal epithelium. The physiological importance of this exchanger is highlighted by the embryonic lethality of NCX1 null-knockout mice. In heterozygous mice, expression of NCX1 protein in the tubular epithelial cells and Ca 2 + influx via NCX1 in renal tubules are markedly attenuated. The second transporter involved in basolateral Ca 2 + extrusion is a P-type ATPase, a high-affinity Ca 2 + efflux pump PMCA1b, which is abundantly expressed in the intestine. Calcium is expelled through a channel-like opening formed by the transmembrane elements, and phosphorylation is believed to bring about the necessary conformational change such that Ca 2 + bound to the protein is propelled through the opening. Similar to NCX1, PMCA1b null knockout in mice is embryonically lethal.
Four PMCA isoforms have been identified to date and alternatively spliced transcripts have been detected ; PMCA1b is however the predominant isoform in the gut, where it is abundantly expressed in the small intestine. Several studies indicated that PMCA1b is positively regulated by 1,25(OH) 2 D 3 in the intestine to increase Ca 2 + absorption. Northern blot analysis indicated that repletion of vitamin D-deficient chickens with 1,25(OH) 2 D 3 increases PMCA mRNA expression in the duodenum, jejunum, ileum, and colon. Additional studies by Johnson and Kumar demonstrated that 1,25(OH) 2 D 3 causes an increase in the abundance of PMCA protein and stimulates Ca 2 + extrusion. However, plasma 1,25(OH) 2 D 3 was not found to be correlated with PMCA1 expression in humans. PMCA activation is dependent upon CaM and inhibition of CaM is, in turn, known to prevent PMCA stimulation. Although some data suggest that extrusion is not the rate-limiting step for Ca 2 + absorption, the estimated V max of PMCA1b has been reported to be in the range of 20–30 nM Ca/min/mg protein , which should be adequate to extrude Ca 2 + even at the highest rates of Ca 2 + transport.
59.3.2.5
Vesicular Transport Model
According to this model, apical formation of Ca 2 + loaded vesicles is initiated upon TRPV-mediated Ca 2 + uptake. These vesicles can then be transported vectorially by microtubules, or they can fuse with endoplasmic reticulum followed by passive diffusion within the ER lumen. Ca 2 + is then released from the ER at the basolateral membrane through calcium release channels and extruded by NCX1 and PMCA1b (see below). Alternatively, these vesicles may fuse with lysosomes, which move laterally to eventually coalesce with the lateral membrane and release the content through exocytosis. This route is also stimulated by 1,25(OH) 2 D 3 . Net epithelial Ca 2 + is inhibited by chloroquine, suggesting that this may be the main route of transcellular Ca 2 + trafficking.
59.3.2.5.1
Vesicular Transport: Apical Entry
Secondarily to TRPV5/6-mediated Ca 2 + influx, the rapid increase in Ca 2 + concentration around the apical region can disrupt the actin filaments near the Ca 2 + channels and initiate the formation of Ca 2 + -enriched endocytic vesicles. It is also possible that Ca 2 + influx promotes transitory insertion of P-type calcium channels in the surface membrane, which is then followed by compensatory endocytosis and formation of vesicles containing Ca 2 + transporters. Indeed, both TRPV5 and TRPV6 have been found to colocalize with a marker of recycling endosomes Rab11a in renal epithelial cells. A third possibility is direct filling of a vesicle by the Ca 2 + channel. Ultimately, the newly formed vesicles are transported vectorially by microtubules.
59.3.2.5.2
Vesicular Transport: Transcellular Ca 2 + Movement and Basolateral Ca 2 + Exit
An earlier study by Warner and Coleman utilizing X-ray probe analysis in intestinal epithelial cells found discrete localizations of Ca 2 + under transport conditions rather than a diffuse cytoplasmic presence. Subsequently, Davis et al., working with chick duodenum, identified these vesicles as lysosomes. The same group later showed that in 1,25(OH) 2 D 3 -treated rachitic chicks there was an increased Ca 2 + concentration and lysosomal count in intestinal epithelial cells. These vesicular/lysosomal structures were membrane bound, enriched in the lysosomal marker acid phosphatase, had a high Ca 2 + content, moved laterally, and eventually coalesced with the lateral plasma membrane, leading to the exocytosis of their contents. The involvement of lysosomes in transcellular Ca 2 + transport in intestinal epithelia was confirmed in several other studies (for review see Ref. ). According to the vesicular transport model, exocytosis delivers Ca 2 + to the basolateral membrane of the polarized intestinal epithelium. Indeed, lysosomotropic agents such as chloquine, quinarine, or ammonium chloride inhibit the net duodenal transport of Ca 2 + . Moreover, Ca 2 + transport is also accompanied by secretion of lysosomal cathepsin B.
59.3.2.6
Tunneling Through the Endoplasmic Reticulum
This model of transcellular Ca 2 + transport was demonstrated in pancreatic acinar cells. Although not confirmed experimentally, this route may also contribute to vectorial Ca 2 + transport in intestinal epithelial cells. According to this model, Ca 2 + enters the cell through channels as in the preceding models and is transported to the basolateral membrane through passive diffusion in the endoplasmic reticulum. This process involves active Ca 2 + buffering rather than the passive buffering that has been described for facilitated diffusion. Ca 2 + is extruded to the interstitium via basolaterally expressed Ca 2 + -ATPases and Na + /Ca 2 + exchangers. It has been hypothesized that the endoplasmic reticulum buds off transport vesicles filled with calcium. Fig. 59.2 depicts the three models of transcellular Ca 2 + transport discussed above. Although still little is known about the relative contribution of each mechanism in the transepithelial Ca 2 + transport in the intestine, it is likely that all three models function in a coordinated fashion to provide the necessary redundancy aimed at meeting the short- and long-term systemic Ca 2 + requirements.

59.3.3
Regulation of Intestinal Calcium Absorption
59.3.3.1
Dietary Calcium
The importance of adequate calcium intake has been best illustrated by studies utilizing VDR and 1α-hydroxylase-knockout mice. The skeletal phenotype of VDR-null mice can be completely rescued by feeding the animals a diet high in calcium, phosphorus, and lactose. Additionally, the abnormal phenotype of 1α-hydroxylase-knockout mice can be corrected by high dietary Ca 2 + intake. The expression of calcium transport proteins was subsequently studied in these knockout models, so as to be able to distinguish the effects of hypocalcemia from those of vitamin D deficiency. Thus studies were initially performed in 1α-hydroxylase-ablated mice fed normal versus high Ca 2 + rescue diets. It is known that under normal circumstances, increased plasma calcium levels act via a negative feedback loop to stimulate PTH secretion, which eventually suppresses 1α-hydroxylase activity, which in turn decreases renal 1,25(OH) 2 D 3 production and calcium transport in the intestine. This seems to be mediated by the expression of the intestinal calcium transport proteins TRPV6, calbindin-D 9k , and PMCA1b, which was normalized by feeding the knockout mice the high calcium rescue diet. Comparable observations were made in VDR-knockout mice where duodenal TRPV6 mRNA levels were induced by dietary calcium. Studies with both of these animal models revealed that calcium supplementation can increase transcriptional initiation of genes encoding calcium transporter proteins in the absence of circulating 1,25(OH) 2 D 3 , but the molecular mechanism of this vitamin D-independent calcium sensitive pathway remains elusive. It is, however, likely that in addition to the 1,25(OH) 2 D 3 response elements in the promoter regions of the TRVP6 and the calbindin-D 9k genes, calcium responsive cis -acting elements are also present. Several promoter elements have been proposed to function as calcium-sensitive transcriptional modulators, including the serum-responsive element in the cAMP/calcium responsive element. However, detailed promoter studies are necessary to characterize fully any putative calcium-responsive elements in the genes responsible for transepithelial calcium transport in the small intestine.
59.3.3.2
Intracellular Ca 2 +
The Ca 2 + concentration in the intestinal lumen can vary greatly, and the sudden appearance of high Ca 2 + level in the lumen can be disastrous, as sustained increases in intracellular Ca 2 + may cause cell death. Thus, Ca 2 + -transporting cells express calbindins to buffer the increases in intracellular Ca 2 + , resulting from Ca 2 + entry through TRPV6 channels. Additionally, to avoid elevations in intracellular Ca 2 + to toxic levels beyond the buffering capacity of the calbindins, TRPV6 exhibits Ca 2 + -dependent inactivation. It has both a fast phase (within 50 ms) and a slow phase of inactivation (over ~ 1 s). The slow phase involves direct binding of calmodulin (CaM) to the TRPV6 C-terminal region. Ca 2 + -dependent binding of CaM to human TRPV6 inactivates the channel, via a protein kinase C site present within the CaM-binding site. The phosphorylation of this protein kinase C site prevents CaM binding, thereby maintaining the activity of TRPV6, so as to allow more Ca 2 + to enter the cell. The first intracellular loop of TRPV6 determines the fast inactivation, which may involve direct interaction of calcium with the channel on the cytoplasmic side of the membrane. In addition, H587, a positively charged amino acid residue in the last transmembrane segment of TRPV6, has also been identified as being involved in fast inactivation.
This feedback inhibition of TRPV6 activity accomplishes two objectives: (1) it protects the cell from Ca 2 + overload and (2) it also limits the flow of Ca 2 + into the cell. TRPV6 activity is inversely related to the intracellular Ca 2 + level on its cytoplasmic side. Thus, the availability of calbindin-D 9k to buffer the local increase in Ca 2 + near the apical membrane will reduce the Ca 2 + -dependent feedback inhibition, and in turn increase the movement of Ca 2 + into the cell. Therefore, a coordinated increase in the expression of both the apical channel TRPV6 and intracellular calbindin-D 9k is necessary to achieve maximal Ca 2 + influx across the apical surface of intestinal epithelial cells. The observation of a lag period in the calbindin-D 9k response to 1,25(OH) 2 D 3 relative to that of TRPV6 suggests that the increase in calbindin-D 9k is an “amplifying” mechanism that increases Ca 2 + influx, both by relieving feedback inhibition of the apical entry step and by facilitating diffusion of Ca 2 + from the apical to the basolateral membrane. The time lapse in calbindin-D 9k production and response to 1,25(OH) 2 D 3 administration suggests that the increase in intracellular Ca 2 + as a result of TRPV6 expression could contribute to calbindin induction.
59.3.3.3
1,25(OH) 2 D 3
The biochemistry, nutritional aspects, metabolism, and physiological and therapeutic potential of vitamin D and its analogs have been the subject of countless reviews and monographs. Most recent and most comprehensive reviews can be found in the recent edition of M.F. Holick’s “Vitamin D: Physiology, Molecular Biology and Clinical Applications” and in the chapter on vitamin D in this book by Wesley Pike and colleagues.
Vitamin D 3 (cholecalciferol) is supplied from the diet, mostly from fortified dairy products and fish oils, or is synthesized in the skin as previtamin D 3 from 7-dehydrocholesterol by ultraviolet irradiation. Previtamin D 3 spontaneously, but slowly isomerizes to vitamin D 3 (cholecalciferol), which is then transported in the blood by the vitamin D-binding protein (DBP) to the liver, where it is hydroxylated at C-25 by one or more cytochrome P450 vitamin D 25-hydroxylases (CYP2R1, CYP2D11, and CYP2D25), resulting in the formation of 25-hydroxyvitamin D 3 (25(OH)D 3 ). Due to its stability and ease of assay, 25(OH)D 3 is almost exclusively used clinically as a measure of vitamin D status. 25(OH)D 3 is transported by DBP to the kidney, where it is endocytosed in the proximal tubules and is further hydroxylated at position C-1 by 1α(OH)ase, resulting in the hormonally active form of vitamin D, 1,25-dihydroxyvitamin D 3 (1,25(OH) 2 D 3 ), which is responsible for most, if not all of the biologic effects of vitamin D. 24-Hydroxylase (CYP24, 24(OH)ase), a renal mitochondrial P450 enzyme, can hydroxylate both 25(OH)D 3 and 1,25(OH) 2 D 3 , with preference for the latter. Catabolism of 1,25(OH) 2 D 3 to 1,24,25(OH) 3 D 3 decreases its biological activity, while hydroxylation of 25(OH)D 3 to 24,25(OH) 2 D 3 leads to a reduction in the pool of 25(OH)D 3 available for 1α-hydroxylation. Therefore, 24(OH)ase functions to inactivate vitamin D.
Vitamin D plays a key role in skeletal and mineral homeostasis. It is frequently viewed as a pro-bone anabolic hormone due to its positive effects on intestinal and renal Ca 2 + and P i (re)absorption and through its positive effects on osteoblast differentiation and bone matrix synthesis. Renal synthesis of 1,25(OH) 2 D 3 depends upon the Ca 2 + status of the body. When Ca 2 + intake is sufficient and the plasma Ca 2 + concentration is normal, renal 1α-hydroxylase activity is low because there is no need for additional body Ca 2 + . However, when body Ca 2 + levels are low, dietary calcium absorption is low and the plasma Ca 2 + concentration falls, the activity of this enzyme increases to produce the active metabolite 1,25(OH) 2 D 3 , to ensure that additional Ca 2 + will be absorbed from the gastrointestinal tract. The molecular mechanism of 1,25(OH) 2 D 3 regulation of intestinal calcium transport likely involves the direct interaction of 1,25(OH) 2 D 3 with the nuclear vitamin D receptor (VDR). The genomic mechanism of action is similar to that of other steroid hormones, and is mediated by stereospecific interaction of 1,25(OH) 2 D 3 with the VDR, which heterodimerizes with the retinoid X receptor (RXR). The heterodimerized receptor then binds to a vitamin D-response element (VDRE) in the promoter of target genes, and transcriptional initiation occurs via an interaction of the VDR with coactivators and the transcriptional machinery. VDR is mainly expressed in epithelia that a play a role in Ca 2 + (re)absorption, confirming the importance of the genomic actions of 1,25(OH) 2 D 3 for body calcium homeostasis. Although transcriptional mechanisms of vitamin D-mediated Ca 2 + absorption received considerably more attention, posttranscriptional (nongenomic) mechanisms have also been reported as mediating Ca 2 + apical entry, cytoplasmic diffusion, and paracellular transport.
While the majority of the discussion presented blow is related to the effects of the more biologically active 1,25(OH) 2 D 3 , it is not quite clear whether intestinal Ca 2 + absorption is responsive solely to this metabolite, or if it can also be positively affected by an improved vitamin D status as measured by serum 25(OH)D 3 independent of renal conversion to 1,25(OH) 2 D 3 . Heaney et al. found that Ca 2 + absorption in healthy humans was increased by 25% after 4 weeks of treatment with 50 μg 25(OH)D per day without concomitant changes in serum 1,25(OH) 2 D 3 levels. These results could be explained by extrarenal expression of 1α hydroxylase (CYP27B1), which is also present in the intestinal mucosa. However, several other studies cast doubt on role for high vitamin D status as a regulator of intestinal calcium absorption by describing no significant association between 25(OH)D 3 level and higher Ca 2 + absorption efficiency, or showed only a very modest positive relationship.
Catabolic hydroxylation of 25(OH)D 3 to 24,25(OH) 2 D 3 by 24(OH)ase has been typically viewed as leading to a reduction in the pool of 25(OH)D 3 available for 1α-hydroxylation. However, 24,25(OH) 2 D 3 , made under conditions of vitamin D sufficiency, also acts as an endogenous inhibitor of both 1,25(OH) 2 D 3 and PTH-stimulated calcium transport. The mechanism of this phenomenon has been reviewed by Khanal and Nemere. 24,25(OH) 2 D 3 is believed to bind catalase and decrease its activity thus leading to elevated H 2 O 2 levels. Peroxide in turn downregulates the 1,25D 3 -MARRS receptor (but not the VDR) as well as protein kinase C signaling pathway.
59.3.3.3.1
The Effects of 1,25(OH) 2 D 3 on Paracellular Intestinal Ca 2 + Transport
Vitamin D signaling has been shown to increase diffusional Ca 2 + flux across the intestine, particularly in the jejunum and ileum. More recently, in studies with rat duodenum in Ussing chambers, 1,25(OH) 2 D 3 was shown to induce Ca 2 + absorption through a solvent drag mechanism that could be inhibited by PI3K, PKC, and MEK antagonists. Interestingly, 1,25(OH) 2 D 3 -altered Ca 2 + ion movement and transepithelial electrical resistance (TEER) but not mannitol flux, an observation suggesting a vitamin D-induced change in the tight junction charge selectivity and perhaps tight junction composition. Indeed, as described earlier in this chapter, 1,25(OH) 2 D 3 significantly increased claudin-2 and -12 transcript levels in Caco-2 cells. Moreover, mRNA and protein levels for these two claudins were significantly lower in the jejunum of VDR knockout compared to wild-type mice, and Ca 2 + permeability was reduced by siRNA-mediated knockdown of claudin-2 and -12. The latter study did not address, however, whether the claudin-mediated changes in 1,25(OH) 2 D 3 -induced Ca 2 + flux represent the saturable or nonsaturable component of Ca 2 + transport. This observation, on the other hand, is in agreement with an older study by Sheikh et al. who found that the nonsaturable component of ileal Ca 2 + absorption was reduced in patients with chronic renal disease associated with low serum 1,25(OH) 2 D 3 . Moreover, claudin-2 and -12 expression is higher in the jejunum and ileum, which would indicate that the stimulatory effects of 1,25(OH) 2 D 3 on paracellular Ca 2 + transport may be limited to these segments of the gut, while the effects of 1,25(OH) 2 D 3 on the saturable component (facilitated diffusion) take place primarily in the duodenum (see Sections 59.3.3.3.2–59.3.3.3.6 ).
59.3.3.3.2
The Effects of 1,25(OH) 2 D 3 on Facilitated Ca 2 + Diffusion: Apical Ca 2 + Entry
Several studies consistently indicated that the expression of TRPV6 is tightly regulated by 1,25(OH) 2 D 3. This was first explored in Caco-2 cells, in which the induction of TRPV6 by 1,25(OH) 2 D 3 was more robust than that of calbindin D 9k and PMCA1b, and occurred several hours sooner. Regulation of TRPV6 by 1,25(OH) 2 D 3 was later confirmed in a study using vitamin D receptor null mice. Duodenal TRPV6 mRNA was reduced ~ 90%, and intestinal calcium absorption was reduced threefold in two VDR-knockout strains fed a normal Ca 2 + diet. Calbindin D 9k was decreased in only one strain of VDR-null mice, and expression of the plasma membrane Ca 2 + -ATPase (PCMA1b) was normal in both strains. These data indicate that the decrease in TRPV6 expression is responsible for the decrease in Ca 2 + absorption seen in these mice. Thus, these two studies demonstrate that out of the known calcium transport-related genes, TRPV6 is the most robustly regulated by vitamin D.
Conversely, TRPV6 mRNA levels were not significantly correlated with vitamin D metabolite levels, but were moderately correlated with calbindin D 9k , and more strongly correlated with PMCA1b in human duodenal biopsy samples from 20 normal subjects. However, interpretation of the results of this study may have been compounded by the varying ages and uncontrolled Ca 2 + intake of the subjects, a factor that was later found to have a significant impact on TRPV6 expression. These studies showed that TRPV6 expression was greatly reduced on a high Ca 2 + diet, and greatly increased on a low Ca 2 + diet, and the extent of the reduction was independent of the vitamin D receptor. Also age and gender can significantly alter interpretation of human Ca 2 + absorption-related studies, as demonstrated, for example, by Walters et al. In men, linear regression analysis showed a strong association with between duodenal TRPV6 expression and 1,25(OH) 2 D 3 , which was unaffected by age. In women, however, there was no significant relationship between TRPV6 and 1,25(OH) 2 D 3 , but there was a significant decrease of TRPV6 with age, which closely correlated with the expression of VDR.
In addition to the VDR-knockout mice, 1α-hydroxylase -knockout mice have also been developed to dissect systematically the genetic regulation of calcium transport genes and to determine the functional consequences on transcellular calcium transport. 1α-Hydroxylase-null mice develop distinct histological evidence of rickets and osteomalacia. In these mice, there is a correlative relationship between the expression of TRPV6, calbindin-D 9k , and PCMA1b in the duodenum and serum calcium concentration. Normalization of the plasma calcium concentration by 1,25(OH) 2 D 3 restored the expression level of the calcium transporters, confirming the essential role that these proteins play in active 1,25(OH) 2 D 3 -mediated calcium absorption.
59.3.3.3.3
The Effects of 1,25(OH) 2 D 3 on Facilitated Ca 2 + Diffusion: Transcellular Ca 2 + Movement
The role of calbindin D 9k in transcellular Ca 2 + absorption across the intestinal epithelium was discussed earlier in this chapter. In vitamin D-deficient animals as well as VDR-knockout mice, calbindin levels in the intestine are significantly reduced, and exogenous 1,25(OH) 2 D 3 significantly increases duodenal calbindin expression. These and many other studies point to an important role for vitamin D 3 -inducible calbindin D 9k in intestinal Ca 2 + transport where it acts as an intracellular buffer or as an intracellular binding protein that permits Ca 2 + shuttling away from the apical membrane and toward the basolateral membrane. However, as pointed out earlier, the role of calbindin CBD 9k in not unequivocal. Intestinal Ca 2 + absorption can be low even when calbindin protein levels are elevated and neither basal nor 1,25(OH) 2 D 3 -induced Ca 2 + absorption are affected in CBD 9k null-knockout mice. The response to 1,25(OH) 2 D 3 was blunted only in TRPV6/CBD 9k double knockout mice, suggesting compensatory mechanisms and/or more complex interactions between TRPV6 and calbindin CBD 9k than previously anticipated.
59.3.3.3.4
The Effects of 1,25(OH) 2 D 3 on Facilitated Ca 2 + Diffusion: Basolateral Ca 2 + Extrusion
When Wasserman et al. and later Cai et al. identified the plasma membrane Ca 2 + ATPase 1b (PMCA1b) as responsible for basolateral extrusion of calcium, vitamin D deficiency was shown to lead to decreased PMCA1b expression in the chick intestine. Vitamin D repletion or low Ca 2 + diets increased it by two- to threefold. However, in two distinct lines of VDR-knockout mice, there was no difference in PMCA1b expression under physiological conditions, and a somewhat unexpected decrease in mice fed a low Ca 2 + diet. It is unlikely that this discrepancy is related to species differences as 1,25(OH) 2 D 3 also induced intestinal PMCA1b in 1α-OHase-knockout mice. Although a VDR-independent mechanism is plausible, it remains to be experimentally addressed.
The studies outlined above demonstrate that of the known calcium transport-related genes, TRPV6 is the most dependent on vitamin D regulation. They also suggest that the TRPV6-mediated apical entry step is a rate-limiting step for vitamin D 3 -regulated Ca 2 + transport, rather than the intracellular diffusion step mediated by calbindin D 9k or the extrusion step mediated by PMCA1b.
59.3.3.3.5
The Effects of 1,25(OH) 2 D 3 on Vesicular Ca 2 + Transport
As discussed above, lysosomes play a key role in vesicular Ca 2 + transport in intestinal epithelial cells. 1,25(OH) 2 D 3 has been shown to increase the number of lysosomes in chick intestine as well as the release of lysosomal content from isolated rat enterocytes. There is other indirect evidence supporting the role of vitamin D 3 in vesicular Ca 2 + transport. The level of lysosomal Ca 2 + is increased 3.1-fold within 10 h of 1,25(OH) 2 D 3 treatment.
In the same study with the chick intestine, after 1,25(OH) 2 D 3 stimulation, calbindin D28k was identified in endosomal vesicles and Ca 2 + -containing lysosomes. These findings have not been reproduced in mammalian intestinal epithelial cells and their relevance to vitamin D regulated vesicular Ca 2 + transport is not known.
59.3.3.3.6
Transcaltachia
Transcaltachia was first observed in A.W. Norman’s laboratory using vitamin-D replete chicks and was defined as “the rapid hormonal stimulation of intestinal Ca 2 + transport”. In contrast to the mechanisms described earlier in this chapter, transcaltachia is a mode of Ca 2 + transport that occurs within minutes of exposing intestinal epithelial cells to 1,25(OH) 2 D 3 , is initiated at the cell membrane and involves nongenomic mechanisms such as activating kinase signaling pathways and the opening of chloride channels. Interestingly, in the initial report, transcaltachia was lost in the intestine of vitamin D 3 -deficient chicks. This observation suggested the presence of a basolateral membrane receptor in enterocytes, which was dependent on vitamin D 3 status. One candidate protein to mediate transcaltachia is a novel vitamin D-binding protein called the membrane-associated rapid response steroid-binding protein (1,25D 3 -MARRS). MARRS (also known as ERp57, PLCα, or PDIA3) knockdown lead to a reduced phosphate uptake into chick enterocytes and enterocyte-specific deletion of MARRS in mice resulted in impaired 1,25(OH) 2 D 3 binding and disrupted vitamin D-regulated Ca 2 + uptake. While trancaltachia has good experimental support, its physiological relevance is not without controversy. The conditions required for trancaltachia (rapid serum 1,25(OH) 2 D 3 fluxes under normocalcemic dietary conditions) are unlikely to occur physiologically; therefore, the contribution of this mechanisms to total intestinal Ca 2 + absorption remains unclear.
59.3.3.4
PTH and the Calcium-Sensing Receptor (CaSR)
The parathyroid glands play a critical role in maintaining extracellular calcium concentrations, via the ability to sense minute changes in blood calcium levels. The calcium-sensing receptor (CaR) in parathyroid chief cells is able to sense circulating calcium levels and subsequently to induce release of PTH when physiologically necessary. In response to low blood calcium, PTH is secreted into the circulation, which then activates the PTHR1 receptor predominantly in kidney and bone. This receptor, once activated by interaction with PTH, stimulates the activity of renal 1α- hydroxylase and suppresses renal 24-hydroxylase (CYP24) mRNA thereby indirectly leading to increased 1,25(OH) 2 D 3 -dependent intestinal calcium absorption. Furthermore, immunohistochemical analysis of rat duodenal sections demonstrated localization of the PTHR1 receptor in epithelial cells along the villus, with intense staining of brush-border and basolateral membranes and the cytoplasm. Direct effects of PTH on calcium transport by isolated rat duodenal enterocytes have been also reported. Initial studies utilizing perfusion of isolated duodenal loops showed that PTH administration increased calcium absorption. These findings were later confirmed in studies that demonstrated that PTH significantly stimulates enterocyte calcium uptake. However, despite these recent investigations, molecular mechanisms of direct PTH action in mediating intestinal calcium transport remain poorly understood. While serum PTH levels vary significantly throughout the course of a day (i.e., high in the morning, lower after consuming calcium-rich meals), Ca absorption efficiency has not been shown to follow a similar diurnal rhythm. To directly address the existence and physiological relevance of PTH signaling in intestinal Ca 2 + absorption, a mouse model with targeted deletion of intestinal PTH receptor 1 is needed.
The CaSR thus plays a crucial role in the regulation of calcium homeostasis by sensing minute changes in circulating calcium levels. In the parathyroid gland, the CaSR allows parathyroid cells to detect changes in blood ionized calcium concentration, to modulate PTH secretion accordingly, and thus to maintain serum calcium levels within a narrow physiological range. CaSR forms a unique molecular target for drugs that can directly alter the activity of the receptor, and thereby modulate extracellular calcium balance. Recently, several compounds, including NPS R-467, have been identified that can activate the CaSR and suppress serum PTH and Ca 2 + levels. However, the involvement of intestinal calcium transport in NPS R-467 mediated hypocalcemia is unclear. Thus, a recent investigation sought to determine the effect of this CaSR agonist on the expression of intestinal calcium transport proteins. These investigators infused mice with NPSR-467 continuously for 7 days and noted reduced serum PTH levels, which were accompanied by a significant decrease in serum calcium concentration. Molecular analysis of duodenal samples demonstrated downregulation of mRNA and protein expression levels of proteins involved in active transcellular calcium absorption. It is currently unknown, however, whether the effects of NPSR-467 could involve a direct action of CaSR in the intestine, or whether the acute hypocalcemic response to this and other similar compounds results from the inhibition of PTH secretion.
59.3.3.5
Estrogen
The intestine is a potential site for estrogen action and there is accumulating evidence that estrogen plays a physiological role in the regulation of intestinal calcium absorption. Estrogen receptors are present in the duodenum and colon; however, the underlying mechanism by which estrogen may regulate calcium absorption is still poorly understood. Additionally, it has not been conclusively established whether there is a direct effect of estrogen on intestinal calcium transport or whether it indirectly mediates this process via an effect on vitamin D metabolism.
It has been long known that in postmenopausal women, calcium balance falls significantly as a result of a reduction in calcium absorption and an increase in urinary calcium loss. Estrogen deficiency can reduce serum 1,25(OH) 2 D 3 level, but this can be reversed by estrogen repletion, which is accompanied by increased fractional calcium absorption. This suggested that estrogen depletion leads primarily to a disruption in vitamin D 3 metabolism.
However, more recent findings suggested that TRPV6 expression was directly regulated by estrogen, as duodenal expression of TRPV6 mRNA in 1α-hydroxylase-null mice and ovariectomized rats is increased by 17β-estradiol administration. Also, another recent study demonstrated that intestinal calcium transport was stimulated by 17β-estradiol, independently of circulating 1,25(OH) 2 D 3 levels. It was additionally reported that duodenal TRPV6 expression is reduced in estrogen receptor α-knockout mice and enhanced by estrogen treatment, and further that the estrogen effect on intestinal calcium absorption is mediated by an estrogen receptor (ER). In this investigation, TRPV6 expression was also shown to be enhanced in pregnant VDR-knockout mice and in wild-type littermates. Furthermore, in lactating mice, duodenal TRPV6 expression levels increased 13-fold. Thus, it is clear that the expression of TRPV6 epithelial calcium channels is influenced by estrogen status. Estrogens, hormonal changes during pregnancy, and lactation have distinct vitamin D-independent effects on active duodenal calcium absorptive mechanisms, primarily via a major induction of TRPV6 mRNA expression. These estrogen effects seem to be mediated solely by estrogen receptor α. Interestingly, Weber et al. recently described an estrogen-responsive element in the promoter of the mouse TRPV6 gene ; however, detailed promoter analysis is necessary to identify the regulatory sites involved in estrogen-mediated regulation of TRPV6. Despite these recent investigations, it remains to be conclusively demonstrated that these changes have functional implications on intestinal Ca 2 + absorption.
Overall, these data confirm that estrogen and 1,25(OH) 2 D 3 are independent, potent regulators of TRPV6 expression, which is involved in active intestinal calcium absorption. These data thus suggest that the function of estrogen in the maintenance of body calcium balance could be at least partially fulfilled by regulation of TRPV6 levels, thereby controlling intestinal calcium absorption to maintain normal serum Ca 2 + levels, which are necessary for proper bone calcification.
59.3.3.6
Thyroid Hormone
The thyroid hormones, thyroxine (T 4 ) and triiodothyronine (T 3 ), function primarily as key regulators of metabolism. Several studies have suggested that thyroid dysfunction is also associated with disturbances of calcium and phosphate homeostasis. Hyperthyroidism has been associated with hypercalcemia and high bone turnover rates leading to osteopenia. Consistently, compared to healthy animals, Ca 2 + uptake was higher in duodenal brush-border membrane vesicles (BBMV) isolated from hyperthyroid rats and lower in BBMV from hypothyroid rats. Thyroid hormones increase serum PTH and 1,25(OH) 2 D 3 levels; therefore, their effects on intestinal Ca 2 + absorption may be indirect. T 3 , however, directly affects the developing intestine. Cross and Peterlik showed that although T 3 treatment alone had no effect upon jejunal calcium uptake, it augmented the effect of 1,25(OH) 2 D 3 on Ca 2 + transport. These findings suggest that T 3 may regulate VDR levels in the intestinal epithelium. This, however, has not been proven. Other mechanisms of thyroid hormone-induced sensitization to vitamin D 3 were proposed by Schräder et al. who suggested that synergistic effects of T 3 and 1,25(OH) 2 D 3 on calbindin D 9k gene transcription is mediated via the formation of vitamin D receptor (VDR)-thyroid hormone receptor (TR) heterodimers, which interacts with putative VDREs. However, this observation was not confirmed in another study where the authors showed the opposite effects and that thyroid hormone receptor (TR) expression can repress 1,25(OH) 2 D 3 -induced transcription predominantly by sequestering RXR.
59.3.3.7
Growth Hormone and IGF-1
Denis et al. demonstrated that intestinal calcium and phosphate transport is positively regulated in pigs by exogenous administration of porcine growth hormone (GH). These investigators treated growing pigs for two months with daily injections of growth hormone, and then performed a 10-day balance study at the end of this period. They found that all aspects of mineral homeostasis were stimulated, including circulating 1,25(OH) 2 D 3 levels (inc. by 40%), Ca 2 + absorption and retention (inc. 70%), P i absorption (inc. 33%) and retention (inc. 45%), and jejenal calbindin-D 9k levels (inc. 40%). It is not known however, if these physiological changes noted with exogenous growth hormone treatment are direct effects or if they are principally mediated by effects on vitamin D 3 homeostasis.
During childhood and adolescence, GH and its physiologic mediator insulin-like growth factor I (IGF-1) have a major role in linear bone growth and accrual of bone mass. GH can also promote intestinal Ca 2 + absorption either indirectly, through activation of renal 1α-OHase and increased 1,25(OH) 2 D 3 serum level ;, or directly by stimulating expression of duodenal calbindin D 9k levels without significantly affecting serum 1,25(OH) 2 D 3 levels. Moreover, GH treatment can prevent the decrease in VDR expression in ovariectomized rats, suggesting that GH may increase sensitivity to 1,25(OH) 2 D 3.
At least a part of the effect of GH on Ca 2 + absorption is believed to be mediated through IGF-1 via a vitamin D 3 signaling-independent mechanism(s). In men, Ca 2 + absorption was positively correlated with IGF-1 and that age-related correlation between declines in IGF-1 and Ca 2 + absorption could not be explained simply by a reduction in serum 1,25(OH) 2 D 3 levels. However, the molecular mechanisms by which the GH/IGF-1 axis may influence the intestinal Ca 2 + absorption independently of vitamin D 3 remain unknown.
59.3.3.8
Klotho
In 1997, Kuro-o et al. described transgenic mice obtained by accidental mutational insertion, which exhibited symptoms typical of human aging: short lifespan, infertility, atherosclerosis, skin atrophy, severe osteoporosis, and emphysema. A then unknown gene was disrupted or mutated in its 5′-flanking promoter region by the random insertion of an exogenously introduced transgene. The coding region of this mutated gene termed αKlotho (frequently referred to as just Klotho, KL) was still preserved, but its expression was markedly reduced generating a mouse strain with a strong hypomorphic allele. The Klotho gene encodes a 130-kDa single pass transmembrane protein with β-glucuronidase activity, which is primarily expressed in epithelial cells of the renal distal tubules, the choroid plexus, and parathyroid gland. A soluble form can also be found in blood plasma and cerebrospinal fluid. αKlotho circulates as an endocrine substance with a multitude of effects, some of which are related to its role as a coreceptor for FGF23, a hormone originating primarily in osteoblasts, which is an intrinsic part of Ca 2 + and P i homeostases. Involvement of the Klotho gene in mineral homeostasis was suggested by recent genetic studies which showed that certain allelic variants of Klotho constitute one of the genetic factors influencing bone mineral density in male adults and in postmenopausal women. All this evidence implies that Klotho is a multifunctional protein that regulates phosphate/calcium metabolism as well as aging. Klotho has also been directly implicated in the regulation of transepithelial Ca 2 + transport via modulation of apical entry as well as basolateral Ca 2 + exit. Apical Ca 2 + entry is partially dependent on TRPV5 and TRPV6 channel abundance at the epithelial cell surface. Shuttling of TRPV5 to and from a subapical pool is a key component of this regulation, and high Ca 2 + influx may be obtained by prolonging the channel’s duration at the cell surface before inactivation or internalization. Klotho, through its glucuronidase activity, modulates N-glycosylation of TRPV5 at Asn358 by removal of the capping sialic acid, which enables physical interaction of galectin 1 with TRPV5 on the cell surface and promotes its apical retention. Reduced renal Klotho expression by colitis-associated proinflammatory cytokine IFNγ has been implicated in downstream endocytosis, ubiquitination, and degradation of TRPV5 and urinary Ca 2 + loss. Activating effects of Klotho on TRPV6-mediated Ca 2 + flux have also been shown, although the effects of Klotho on intestinal Ca 2 + absorption has not been demonstrated to date.
βKlotho, encoded by a paralog KLB gene, regulates energy metabolism as the coreceptor for FGF15/19 and FGF21, analogous to the role of αKlotho for FGF23. Both βKlotho and FGF15/19 are expressed in the intestine and together with hepatic βKlotho have been implicated in the control of Cyp7a1 gene expression and bile acid synthesis.
59.3.3.9
Stanniocalcin
In mammals, early studies on stanniocalcin 1 (STC1) cloning and expression pattern suggested that this hypocalcemic factor is expressed in multiple organs including calcium-transporting epithelia, such as intestine, colon, kidney, and placenta. It is also released by the corpuscles of Stannius , specialized organs that are adjacent to and scattered throughout the kidney. Stanniocalcin acts locally in the gut to modulate calcium and phosphate absorption, and overexpression in mice results in high serum phosphate, dwarfism, and increased metabolic rate. In vivo studies using the rat kidney indicated that STC1 may regulate blood calcium by increasing reabsorption of phosphate, and in vitro experiments on mammalian intestine have shown that STC1 can reduce the movement of calcium while increasing absorption of phosphate across the gut. In both models, the predicted net effect would be a decrease in circulating levels of calcium, thereby acting as an antihypercalcemic factor. Furthermore, expression of stanniocalcin 1 is induced by 1,25(OH) 2 D 3, thus suggesting its participation in a form of a negative feedback regulation. Indeed, overexpression of STC in Caco-2 cells downregulated TRPV5 and TRPV6 mRNA and protein expression, and the reverse trend was seen with siRNA-mediated STC1 knockdown, while PMCA1b, NCX1, or VDR remained unaffected. Few studies have addressed the expression or function of stanniocalcin 1 in mammals in vivo, and the expression of STC1 in the epithelial cells is questionable. Thus, further work is necessary before strong conclusions may be drawn regarding the involvement of this hormone in intestinal calcium transport.
59.4
Intestinal Phosphate Transport
Phosphorus makes up approximately 0.5% of the body of a newborn 0.65%–1.1% of an adult. Most phosphate (85% of total body stores) is deposited in bones and teeth, where it associates with calcium as hydroxyapatite; 14% of phosphate is in cells, while phosphate in serum and extracellular fluids accounts for only 1% of total-body content. In most cases, serum phosphate concentration correlates with total-body phosphate content. Phosphorous is not only a major component of the skeleton but is also a mediator of energy transfer and is involved in a wide variety of metabolic reactions in cells. Phosphorus is present in soft tissues as a soluble phosphate ion and is a component of lipids, proteins, carbohydrates, and nucleic acids. Moreover, energy from a variety of metabolic processes derives primarily from the high-energy phosphate bonds of creatine phosphate and adenosine triphosphate (ATP). Because of the critical role of phosphorous as inorganic phosphate (P i ) and phosphate esters in cell physiology, mammals have developed extensive mechanisms for extracting phosphate from the diet and for the conservation of phosphate absorbed by the intestine. As a consequence, plasma phosphate concentrations are maintained within a relatively narrow range. Although the control of plasma phosphate level is not as precisely controlled as that of calcium, body phosphate concentrations are nonetheless modulated by the interplay of a variety of homeostatic mechanisms, as reviewed by our group. The intestine, which is involved in the extraction of P i from dietary sources, plays a principle role in maintaining body phosphate levels. The amount of dietary phosphate absorbed is approximately equal to the amount of phosphate excreted by the kidney ( Fig. 59.3 ).

59.4.1
P i Supply and Bioavailability
Although the precise requirement for phosphorus is unknown, a dietary intake of ~ 700 mg/day is required to maintain positive phosphate balance in adults ( Table 59.1 ). During pregnancy and lactation, this requirement is increased significantly before the age of 18, while later in adulthood, the recommended dietary requirement remains the same. Phosphate is relatively abundant in most foods, so nutritional phosphate deficiency is uncommon. However, P i deficiency does occur commonly in preterm neonates. Approximately 80% of dietary phosphorous is derived from milk products, grains, and meats, with dairy products constituting a major source of dietary phosphate. In human milk, 1 day after birth, the concentration (mean ± SEM) of phosphate is 0.26 ± 0.16 mM, while 4 days later it has increased 6.6-fold to 1.69 ± 0.11 mM. Thus, maternal milk supplies adequate P i to the rapidly growing infant. Furthermore, US diets are replete with food additives contributing a considerable amount of phosphate to the diet, with these additives contributing as much as 30% of the phosphorous of an average adult diet. Approximately 50%–70% of dietary phosphate is absorbed from normal diets and as much as 90% is absorbed when intake is low, with net absorption being essentially proportional to intake. Following a phosphate rich meal, absorption occurs predominately by passive diffusion across the epithelium of the proximal duodenum. Two transport mechanisms exist, passive/paracellular and active/transcellullar (the latter contributing more to total P i absorption at low P i intake levels). Furthermore, under normal conditions, the urinary excretion of phosphate is comparable to the amount absorbed.
With reduced phosphate intake, intestinal absorption by active transport mechanisms is induced primarily by 1,25(OH) 2 D 3. However, evidence suggests that in the suckling and weanling periods, intestinal P i transport is not 1,25(OH) 2 D 3 -responsive. Urinary excretion of phosphate approaches zero during phosphorus deprivation, as the tubular reabsorption approaches 100% of the filtered load. Thus, both the intestine and kidneys are able to respond to restriction in dietary phosphate by enhancing intestinal absorption and renal conservation. Intestinal secretion of phosphate, which normally averages 3 mg/kg b.w./day, is also reduced during dietary deprivation. However, this adaptation occurs very slowly, so that a transient net phosphate deficiency may occur. During fasting, the concentration of phosphate in the small intestinal lumen decreases to ~ 2 mM/L. Sources of endogenous P i include saliva (4.0 mM/L) and gastric, pancreatic and intestinal secretions (1.0 mM/L). Rapid turnover of enterocytes delivers ~ 250 mg of cellular debris into the intestinal lumen each day, which contributes to the endogenous P i pool. Part of this endogenous pool is reabsorbed by the intestine, while the remainder (3–4 mM or 90–120 mg/day) is excreted in the feces.
59.4.2
Molecular Mechanisms of Intestinal Phosphate Transport
Despite the importance of maintaining serum P i levels within a relatively narrow range, nearly 70% of dietary phosphate is absorbed, resulting in transient postprandial increases in serum phosphate concentrations. Homeostasis of serum phosphate appears to be managed primarily within the renal proximal tubule by the type II sodium-dependent phosphate cotransporters NPT2a ( SLC34A1 ) and NPT2c ( SLC34A3 ). In recent years, data demonstrating that regulation of the renal phosphate transporters can adequately maintain systemic phosphate levels, and the extent of clinical consequences resulting from aberrant renal P i reabsorption has somewhat reduced the interest in the regulation of intestinal phosphate absorption. However, newer data on the regulation and importance of the highly regulated intestinal Na + -dependent NaP i -IIb (Npt2b; SLC34A2 ) transporter drew new attention and appreciation of the contribution of intestinal P i transport to systemic phosphate homeostasis.
59.4.2.1
Mechanisms and Sites of Phosphate Absorption
Intestinal phosphate absorption in mammals occurs primarily in the small intestine, with relative absorptive efficiency in duodenum being greater than jejunum, which is greater still than ileum. However, the bulk of phosphate absorption occurs in the jejunum, due to its longer length and increased transit time of the digesta through this gut segment. In experimental rodents, the contribution of proximal and distal small intestinal segments to P i absorption varies by species. In mice, ileal P i absorption is a significant contributor, whereas in rats maximal absorption occurs in the duodenum with very little absorption occurring in the ileum, which is similar to the pattern reported in humans. However, in both rodent species only the jejunum shows an increase in P i absorption in response to 1,25(OH) 2 D 3 treatment.
Early studies of intestinal transport suggest that paracellular transport driven by a passive diffusional process predominates under standard dietary conditions. The 1980s brought a distinction between sodium-dependent and -independent components. Both of these mechanisms contribute to P i transport across intestinal brush-border and basolateral membranes, with each of these membrane domains exhibiting significant differences in affinities and total transport capacity of the sodium-dependent transport process. Intestinal perfusion of the human jejunum demonstrates that active transport of phosphate is maximal at low phosphate intake levels and conversely, that nonsaturable, passive diffusion occurs with high phosphate concentrations. Due to the negative charges in paracellular intestinal phosphate transport channels, simple diffusion across the intestinal mucosa at low phosphate concentrations via this route is unlikely. The highly electronegative interior of the cell (~ 50 − 70 mV) results in a transmembrane potential difference between the interior of the cell and the intestinal lumen. This potential difference functions as a powerful barrier to the transport of negatively charged <SPAN role=presentation tabIndex=0 id=MathJax-Element-1-Frame class=MathJax style="POSITION: relative" data-mathml='H2PO4−’>H2PO4−H2PO4−
H 2 PO 4 −
or <SPAN role=presentation tabIndex=0 id=MathJax-Element-2-Frame class=MathJax style="POSITION: relative" data-mathml='H2PO42−’>H2PO42−H2PO42−
H 2 PO 4 2 −
ions across the brush-border membrane. Whereas <SPAN role=presentation tabIndex=0 id=MathJax-Element-3-Frame class=MathJax style="POSITION: relative" data-mathml='H2PO4−’>H2PO4−H2PO4−
H 2 PO 4 −
ions, which can accumulate to high concentrations at acidic pH in proximal intestinal segments, are more readily transported against this electronegative gradient, <SPAN role=presentation tabIndex=0 id=MathJax-Element-4-Frame class=MathJax style="POSITION: relative" data-mathml='H2PO42−’>H2PO42−H2PO42−
H 2 PO 4 2 −
(the ionic species which predominates at the alkaline pH of the jejunum and ileum) requires an active, energy-dependent process to ensure adequate absorption. 1,25(OH) 2 D 3 stimulates active phosphate transport across both brush-border and basolateral membranes in the small intestine, and we are beginning to understand the molecular mechanisms involved (as discussed below). Furthermore, because the potential difference across the basolateral membrane of intestinal epithelial cells does not inhibit the transport of negatively charged, ionic phosphate molecules out of the cell into the interstitial space and because the P i concentrations in the cell and plasma are ~ 2 and ~ 1.0 mM, respectively, efflux of phosphate across the basolateral membrane likely occurs passively down an electrochemical gradient. The exact mechanism(s) that control transepithelial movement of P i , remain largely uncharacterized, since the overall regulation of this process is complicated by the fact that P i becomes rapidly integrated into various metabolic processes once it enters enterocytes. As such, the rate and control of phosphate absorption are not only a function of the brush-border and basolateral membrane transport processes but it is also dependent on the rate of utilization and esterification of intracellular phosphate.
59.4.2.2
Na + -Dependent P i Absorption: Type I NaP i Cotransporters
Type I transporter group consists of sodium-phosphate cotransporter NPT1 (SLC17A1/NaP i -1), NPT3 (SLC17A2), NPT4 (SLC17A3), and NPT5 (SLC17A4) that belong to the SLC17 transporter family, which also includes lysosomal acidic sugar transporter (sialin; SLC17A5), vesicular glutamate transporters (SLC17A7, SLC17A6, and SLC17A8, respectively), and a vesicular nucleotide transporter (SLC17A9). Although the first known member, NaP i -1 was cloned as a P i transporter, the SLC17 family is now recognized as multifunctional organic anion transporters, or as an anion:cation symporter family. As an example, expression of NPT5 has been identified in the intestine, but it was postulated to facilitate intestinal ureate excretion.
59.4.2.3
Na + -Dependent P i Absorption: Type II NaP i Cotransporters
Intestinal transport of dietary phosphate is dependent upon the activity of the type IIb, sodium-dependent phosphate cotransporter (NaP i -IIb) ( Fig. 59.4 ). This protein is a member of the SLC34 family of solute carriers, which is comprised of three members: NaP i -IIa ( SLC34A1 ), NaP i -IIb ( SLC34A2 ), and NaP i -IIc ( SLC34A3 ). All three have a preference for divalent ( <SPAN role=presentation tabIndex=0 id=MathJax-Element-5-Frame class=MathJax style="POSITION: relative" data-mathml='HPO42−’>HPO42−HPO42−
HPO 4 2 −
) phosphate and are inhibited by phosphonoformic acid (PFA); NaP i -IIa and NaP i -IIb are electrogenic and transport phosphate with a stoichiometry of 3:1 Na + 🙁 <SPAN role=presentation tabIndex=0 id=MathJax-Element-6-Frame class=MathJax style="POSITION: relative" data-mathml='HPO42−’>HPO42−HPO42−
HPO 4 2 −
) whereas NaP i -IIc is electroneutral with a 2:1 Na + 🙁 <SPAN role=presentation tabIndex=0 id=MathJax-Element-7-Frame class=MathJax style="POSITION: relative" data-mathml='HPO42−’>HPO42−HPO42−
HPO 4 2 −
) stoichiometry. NaP i -IIa is expressed in apical membranes of epithelial cells in the renal proximal tubules, in osteoclasts and also in neurons, while NaP i -IIc expression is restricted to the renal epithelium. NaP-IIb ( SLC34A2 ) was originally identified based on EST clones derived from lung tissue and is highly expressed in lung, small intestine, and many other tissues. By immunofluorescence, NaP i -IIb was localized to brush-border membranes of enterocytes along the entire villus length, although autoradiography studies showed that phosphate uptake is restricted to enterocytes in the mid- to upper regions of duodenal and jejunal villi. On western blots, fully glycosylated NaP i -IIb is seen as a band of approximately 110 kDa in mice, however, smaller bands at 75–80 kDa have been reported in rat, brush-border membrane protein samples. Interestingly, in weanling animals, NaP i -IIb is reported to be only partially glycosylated. Thus, the NaP i -IIb cotransporter is strongly expressed in the small intestinal epithelium and has been long thought to be the major, active transport system for dietary phosphate. Null knockout of the mouse NaP i -IIb resulted in early embryonic lethality, which was attributed its normally high expression in the extraembryonic tissues (labyrinthine zone, where embryonic and maternal circulations were in closest contact) and a defect in phosphate absorption from the maternal circulation. To address the role of NaP i -IIb in postnatal life, Sabbagh et al. created an inducible global-knockout model of NaP i -IIb by crossing SLC34A2 fl/fl or SLC34A2 +/fl mice with CMV-CreER T2 mice. In this study, inducible deletion of the SLC34A2 gene led to increased fecal phosphate excretion and hypophosphaturia. The latter was attributed to reduced serum levels of the phosphaturic hormone FGF23 and increased expression of the renal NaP i -IIa protein. NaP i -IIb-deficient mice fed low-phosphate diet absorbed approximately 50% less phosphate than their wild-type littermates after oral phosphate bolus, thus confirming a major role of this transporter in phosphate absorption. In vitro analysis of active phosphate transport in the isolated ileum (the major site of Na + -dependent P i absorption in mice) demonstrated that NaP i -IIb contributed > 90% of total active phosphate absorption. Consistent with this report, intestinal epithelial cell-specific deletion of SLC34A2 gene led to fecal wasting of P i and complete absence of Na/P i cotransport activity in the ileal brush-border membrane vesicles. Interestingly, blood P i concentrations did not change in these mice, likely due to compensatory increase in renal P i reabsorption via elevated NaP i -IIa, as well as due to reduced plasma levels of intact FGF-23, which normally acts to inhibit NaP i -IIa activity in the renal proximal tubules.
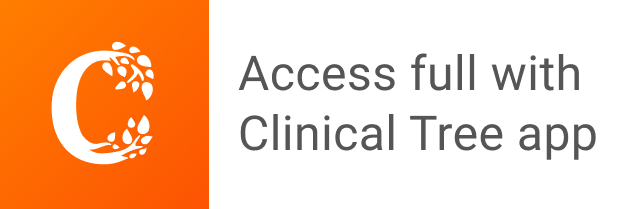