Abstract
Iron derived from dietary sources is essential to support numerous physiological functions in mammals, including oxygen transport, energy production, antioxidant defense, and regulation of gene expression. Absorption of dietary iron in the proximal small intestine is a highly regulated, dynamic process, representing a crucial step in overall body iron homeostasis since humans cannot excrete excess iron. Maintenance of proper tissue iron levels is important given the dire pathophysiologic outcomes associated with iron deficiency (e.g., anemia, lethargy, impaired cognitive development) and iron overload (e.g., oxidative stress, tissue damage, cancer). Iron deficiency is most frequently associated with rapid growth, aging, excessive menstrual blood loss, gastric bypass surgery, and achlohydria. Iron overload, conversely, is usually the result of mutations in genes encoding proteins, which regulate expression of the iron-regulatory hormone, hepcidin, in the liver. Detailed mechanistic investigation of intestinal iron transport is thus critical to develop therapeutic strategies to modulate iron absorption when iron homeostasis is perturbed. This chapter provides updated information regarding recent research on mechanisms of intestinal iron transport, with a focus on human physiology and pathophysiology. Moreover, several controversial areas and unresolved questions will be highlighted throughout, hopefully providing important foci for future investigations.
Keywords
Duodenum, Iron, Nutrition, Absorption, Heme, Divalent metal-ion transporter 1, Ferroportin, Hephaestin, Hepcidin
60.1
Introduction
60.1.1
The Essentiality of Iron and Overview of Intestinal Iron Homeostasis
Iron is abundant in the Earth’s soils and as such, it is ubiquitously present in life forms on this planet. Most species, including humans, require iron for numerous biological functions, yet iron in excess is toxic; as such, iron levels must be precisely managed. Iron is necessary for several critical physiological functions, including oxygen transport, regulation of cell growth and differentiation, energy production, and control of gene expression. Mammals have developed sophisticated regulatory mechanisms that govern iron absorption, storage, and recycling. Iron associates with many proteins and it mediates important functions, including, electron transfer reactions. Some iron-binding proteins have no known enzymatic function, but many others are enzymes, which contain iron as iron-sulfur clusters, heme moieties, or in other molecular configurations. Iron-binding proteins lacking enzymatic activity include those that transport oxygen (hemoglobin and myoglobin). Some iron-sulfur cluster-containing enzymes are involved in energy (i.e., ATP) production via their role in single-electron transfer reactions (e.g., in the cytochromes of the electron transport chain). Heme-containing proteins also participate in a variety of electron transfer reactions in association with various cofactors (e.g., cytochrome P450 complexes). Other proteins transiently bind iron and facilitate its movement across the plasma and intracellular membranes (e.g., divalent metal-ion transporter 1 [DMT1], ferroportin 1 [FPN1). The nonredundant roles of iron in these and other proteins exemplify the importance of iron in mammalian physiology.
Overall body iron homeostasis is controlled by the peptide hormone hepcidin, which functions to decrease serum iron levels by inhibiting the absorption of dietary iron, and iron release from stores in reticuloendothelial (RE) macrophages of the spleen, liver and bone marrow, and hepatocytes. Hepcidin is induced by high body iron content, infection, and inflammation. Conversely, during iron deficiency and hypoxia and when erythropoietic demand increases, hepcidin production is very low, and additional regulatory mechanisms are invoked to increase intestinal iron absorption. Normally, absorption of dietary iron reflects body iron requirements; however, homeostatic dysregulation of iron absorption occurs in numerous disease states, with pathological consequences. Humans have not evolved an efficient iron excretory system, so the overall control of body iron content is ultimately determined by assimilation of dietary iron by the intestine. Absorption of dietary iron typically matches nonspecific losses, which occur via menstrual blood flows, desquamation of skin cells and exfoliation of GI epithelial cells, and in bile and urine. A normal human will absorb 25–50 g of dietary iron over their lifetime. In the adult males, this represents ~ 1 mg of iron/day, while adult females absorb 1.5–2 mg/day to compensate for iron losses associated with menstruation, pregnancy, and lactation. Furthermore, although some species can excrete greater quantities of iron than humans (e.g., rodents), the same basic regulatory schemes that have been described in man appear to operate in other mammals as well.
Physiological studies of iron absorption have been carried out for many decades, but it is only within the last 20 years that the application of molecular and genetic techniques has led to an understanding of basic mechanistic details regarding the transfer of iron across the intestinal epithelium. Anatomically, iron is absorbed predominantly by intestinal epithelial cells (IECs) in the duodenum and proximal jejunum. Absorption is restricted to the differentiated enterocytes on the upper half of the villus. Most dietary iron exists in the Fe 3 + , or ferric form, which is highly insoluble. Ferric iron must thus be reduced to the more soluble ferrous form (Fe 2 + ) before it can be efficiently absorbed. One possible brush-border membrane (BBM) ferrireductase is duodenal cytochrome b (DCYTB), although other candidate proteins have also been identified. Moreover, dietary (e.g., ascorbic acid) and endogenous (e.g., gastric acid) factors also promote reduction of ferric iron. Once reduced, ferrous iron is transported across the BBM via DMT1. The fate of iron within the enterocyte is determined by body iron status. If iron stores are replete, most absorbed iron will be stored within ferritin and later lost when enterocytes are exfoliated from the villus tip. However, if body iron stores are low, iron will be transported out of the cell across the basolateral membrane (BLM) via the iron exporter FPN1. The export (or transfer) process requires that iron be oxidized for binding to transferrin (TF) in the interstitial fluid and plasma, and this likely occurs via the iron oxidase (i.e., ferroxidase [FOX]) hephaestin (HEPH), which is expressed on the basolateral surface of enterocytes. Iron export from duodenal enterocytes is, in part, regulated by the liver-derived, peptide hormone hepcidin, which binds to FPN1 causing its internalization and degradation. Hepcidin expression in hepatocytes is regulated by physiological signals that are transduced to the HAMP gene (encoding hepcidin) by the hemochromatosis (HFE) protein, transferrin receptor 2 (TFR2) and hemojuvelin (HJV), among others. Mutations in the genes encoding these proteins lead to impaired hepcidin production, resulting in inappropriately increased intestinal iron absorption and consequent systemic iron loading, eventually resulting in tissue and organ damage and associated pathological phenotypes. Each of these steps in iron absorption and regulatory mechanisms will be considered in greater detail in subsequent sections.
Iron homeostasis in undifferentiated enterocytes of the intestinal crypts is quite different from what occurs in their mature counterparts. These immature epithelial cells are not specialized for vectorial iron (or nutrient) transport and act rather more like a generic cell. They are rapidly growing and dividing, so they have a high demand for iron, which they absorb as TF-bound iron from the serosal side, unlike mature (i.e., fully differentiated) enterocytes which get much of their iron from dietary components on the luminal side. Both transferrin receptor 1 (TFR1)-dependent and TFR1-independent diferric TF uptake have been demonstrated in cryptal enterocytes. As the cells differentiate and migrate upward along the villus, they take on an absorptive phenotype and subsequently lose the capacity to absorb iron from the circulation.
Disturbances in intestinal iron absorption can have significant clinical consequences. In humans, the most common pathological condition that perturbs iron absorption is hereditary hemochromatosis (HH). HH is a group of genetic diseases in which hepcidin production is impaired leading to dysregulated (and increased) intestinal iron absorption and iron release from stores, eventually resulting in tissue iron overload and associated damage. The most common form of HH results from mutations in the gene encoding HFE, but several less common forms have also been described. Pathological conditions associated with reduced iron absorption, though less common, are still important from a clinical perspective. Impaired iron absorption frequently occurs in patients with malabsorptive disorders, such as celic disease or inflammatory bowel disease (IBD), in individuals who have undergone gastric bypass surgery for morbid obesity, in those who take proton-pump inhibitors for chronic gastric reflux, and in older adults with achlorhydria. Iron-refractory anemias, resulting from impairments in iron absorption, have also been described, but to date, only one of these has been attributed to an intestinal iron transport molecule. These clinical disorders will be considered in greater detail below.
60.1.2
Chapter Overview
This chapter will describe the molecular details of intestinal iron absorption, a process not fully understood, but which is being actively investigated by a large number of groups around the world. The brief overview of the importance of iron as a nutrient and of the basics of the intestinal transport process presented above will be expanded upon in subsequent sections. Dietary and endogenous factors that affect the efficiency of intestinal iron absorption will be considered, along with physical and morphological attributes of the GI system that also regulate this process. The steps involved in transferring iron across the BBM, through the cytoplasm and out of the enterocyte for loading onto transferrin will be delineated in detail. How this complex process is altered during development, both pre- and postnatally, will be outlined. Moreover, systemic and local control of intestinal iron absorption will be considered, as well as how this process is either primarily or secondarily perturbed in various disease states.
60.2
The Anatomy of Iron Absorption—Specialization of the Proximal Small Intestine
Most iron is absorbed in the proximal small intestine, mainly by the duodenum and proximal jejunum. Small amounts of iron may also be absorbed from the stomach, ileum, and colon, but the relative contribution of these sites to overall iron absorption is probably small. The more efficient absorption in the proximal small bowel may be partly due to higher concentrations of luminal iron and a lower pH (which promotes iron reduction), but a number of studies suggest that it is an intrinsic property of the gut mucosa in this region. Recent studies demonstrating higher expression of iron transport proteins (e.g., DCYTB, DMT1, FPN1) in this region of the gut may provide a molecular explanation for these observations. At the level of the intestinal epithelium, iron absorption occurs through the differentiated enterocytes of the mid- and upper villus, rather than across the undifferentiated cells lower on the villus and in the crypts. It is these differentiated enterocytes that express the critical molecules of intestinal iron transit (e.g., DMT1, FPN1, HEPH). Finally, there is accumulating evidence that during the development of iron deficiency there is morphological adaptation of the intestinal mucosa to increase the effective absorptive area and upregulate the capacity of the intestine to absorb iron. For example, in hemolytic anemia, villus enterocytes from lower portions of the villus participate in absorption, during pregnancy villus size is increased, and during iron deficiency, absorption occurs in more distal gut segments and villus width and length are increased and mitosis increases in the intestinal crypts.
60.3
Dietary and Other Factors Which Influence Iron Absorption
Iron is found in the diet in a wide variety of foods in two distinct forms, heme and nonheme (or inorganic) iron. Heme iron is derived from hemoglobin and myoglobin, which are found predominantly in meat products. Heme iron absorption is efficient and mostly unaffected by other dietary constituents. In contrast, nonheme iron, which derives from both plant and meat sources, is highly insoluble (as it exists mainly in the ferric form) and its bioavailability is affected by various dietary components. This is due to the fact that nonheme iron is not sequestered within a stable complex like heme iron, which makes it more reactive and free to interact with other molecules. Dietary and luminal factors, including gastric acid, ascorbic acid, and citrate, help keep inorganic iron in a more soluble, reduced state, and thus in the absorbable (ferrous) form. Decreased gastric juice production or decreased gastric acidity affect the reduction of insoluble ferric iron to soluble ferrous iron and thus may reduce the availability of absorbable iron. In addition to the positive effect of the low pH environment resulting from gastric HCl production, there also exists an acidic microclimate near the apical surface of enterocytes in the “unstirred” water layer. This is produced by the action of an apically expressed sodium‑hydrogen exchanger (NHE), notably NHE3, which exchanges extracellular Na + for intracellular H + . The resulting electrochemical H + gradient from outside to inside the cells serves as the driving force for the intestinal iron transporter, DMT1, which is a ferrous iron/proton cotransporter. This acidic microclimate thus likely has a positive influence on iron absorption. Another factor that has been shown to enhance iron absorption is the consumption of prebiotics, which are indigestible carbohydrates that are fermented by gut bacteria.
Furthermore, there also exists ample evidence that certain dietary factors, mainly derived from plant sources, negatively affect iron absorption. These include phytate, polyphenols, and tannins, which all tightly bind ferric or ferrous iron and decrease their bioavailability. Additional influences on iron absorption include chronic use of proton-pump inhibitors, which reduce gastric acid production and possibly iron bioavailability, alterations in intestinal motility, infection with Helicobacter pylori and mucosal pathologies such as celiac disease. Some of these are considered in more detail below.
60.3.1
Other Influences on Intestinal Iron Absorption
60.3.1.1
Intestinal Transit Time
As mentioned above, the proximal small intestine (duodenum and upper jejunum) is the major site of iron absorption. Given this limited area for absorption, variations in the rate that the digesta transit through the small intestine may contribute to altered intestinal iron uptake. Few studies, however, have measured iron absorption specifically relating to variable GI transit times. One such study utilized rats treated with metoclopramide (oral or I.M.) to reduce transit time or loperamide (orally) to increase transit time. Neither increasing or decreasing intestinal transit time altered the efficiency of Fe absorption, as assessed by whole-body retention of 59 Fe 7 days after a test meal, as compared to vehicle-treated, control rats. Moreover, conditions that decrease the available surface area for nutrient absorption (e.g., short gut syndrome, bariatric surgery), or hasten gastric emptying (infectious or inflammatory diarrhea) thus decreasing transit time, may prevent adequate levels of iron absorption. In addition to shortening transit time, some malabsorption syndromes induce marked inflammation (e.g., IBDs, celiac disease, etc.) and subsequent anemia. Anemia of inflammation (AI) is the result of decreased iron absorption and iron retention in the RE system triggered by proinflammatory cytokine-mediated upregulation of hepcidin expression (as discussed in subsequent sections). Thus, decreased transit time during chronic inflammation may exacerbate the impaired iron absorption that accompanies hepcidin-induced anemia.
60.3.1.2
Helicobacter pylori Infection
Despite its decline in recent years, Helicobacter pylori ( H. pylori) infection remains prevalent worldwide. Multiple studies and metaanalyses have identified a distinct link between H. pylori infection and iron-deficiency anemia (IDA), suggesting that the bacteria may increase iron loss and impair iron absorption. Although the physiological mechanism(s) behind H. pylori -induced anemia remain to be elucidated in detail, it could relate to induction of hepcidin expression in infected individuals, perhaps even in gastric parietal cells. Another proposed mechanism by which H . pylori colonization may decrease iron absorption is through alteration of gastric pH. Specifically, H . pylori infection of the gastric mucosa causes changes in gastric physiology that can lead to atrophic gastritis. Subsequently, the loss of parietal cells due to atrophy causes hypo- or achlorhydria. Under physiological conditions, the acidic pH of the stomach promotes the reduction of ferric iron (insoluble form) to ferrous iron, the more soluble form transported by DMT1. An increase in gastric pH could thus potentially reduce the amount of absorbable ferrous iron. Furthermore, the reduction of ferric iron to ferrous iron is enhanced by dietary and tissue ascorbic acid. Indeed, studies have shown significantly decreased ascorbic acid levels in the mucosa of H. pylori -infected subjects when compared to uninfected individuals, which could potentially exacerbate iron malabsorption. Recent studies have also speculated that H. pylori competes with its host for available iron. Iron is an essential nutrient for bacterial growth and is required for bacteria to retain virulence. Thus, increased iron utilization by bacteria to support their growth may cause iron depletion in the host during H. pylori infection. The mechanism by which H. pylori acquires iron remains unknown, but in vitro studies suggest that the bacteria can disturb host cell polarity and disrupt intracellular iron homeostasis.
60.3.1.3
The Gut Microbiome
Iron is an essential mineral for the survival and growth of numerous cell types, including IECs, and also for commensal bacteria. Host intestinal cells and gut bacteria share a unique symbiotic relationship characterized by continuous cross-communication and subsequent mutually beneficial adaptive changes that ensure their survival. Given the complex physiology of the GI tract, this interconnectedness between host cells and gut bacteria could potentially affect a variable number of GI functions, including iron absorption. It is well established that dietary iron intake levels directly affect the composition of the gut microbiota ; however, the effects of the gut microbiota on iron absorption remain speculative. A recent study showed an 8- to 10-fold increase in intestinal DCYTB and DMT1 protein expression and a twofold reduction in FPN1 expression in the duodenum of germ-free (GF) mice, as compared to GF mice colonized with gut bacteria. Ferritin expression was strongly induced in the colon after bacteria were introduced into the GI tract. The authors suggested that the enterocytes of GF mice were depleted of iron and that upon bacterial colonization, the epithelial cells favor iron storage. Similar studies using germ-free models have shown that the gut microbiota affect many other host intestinal processes, including absorptive, immune, and secretory functions ; however, factors such as rate of colonization and the specific bacterial species present can significantly alter the resulting physiological response. Potential pathological alterations of the gut microbiota should be taken into consideration during iron supplementation or when managing iron-related pathologies, but further research is required to elucidate the mechanisms by which the gut microbiota may influence intestinal iron absorption.
60.4
Iron Transport Across the Enterocyte
60.4.1
General Characteristics of Iron Absorption
Iron absorption occurs through the differentiated epithelial cells (enterocytes) of the mid and upper villus, and predominantly in the proximal part of the small intestine. The proteins involved in this process are listed and briefly described in Table 60.1 . The immature enterocytes of the intestinal crypts handle iron quite differently from their mature counterparts (as noted above). They are not absorptive in nature and take up a large amount of TF-bound iron from the blood, unlike mature enterocytes. As the cells mature and differentiate, they acquire their absorptive characteristics but lose the capacity to take up iron from the circulation. Iron metabolism in crypt cells will not be considered further here.
Protein | Function |
---|---|
DCYTB a | Ferric iron reduction for absorption across BBM; other reductases may exist and could also play a role in this process |
DMT1 | Cotransport of ferrous iron and H + into enterocytes; also transports manganese and possibly other divalent metal ions |
FLVCR | Heme export across BLM into circulation |
FPN1 | Ferrous iron exporter on basolateral surface of enterocytes; hepcidin receptor |
Ferritin | Intracellular iron storage, including within enterocytes; dietary form of iron |
HCP1 | Possible heme importer on apical surface of enterocytes |
HEPH | Oxidation of exported iron for binding to transferrin and distribution to body cells and tissues in blood; expressed on basolateral surface of enterocytes |
HO | Oxidation of heme molecule to release ferrous iron in endosomes |
Hepcidin | Liver-derived, peptide hormone that inhibits intestinal iron export by promoting internalization and degradation of FPN1 |
LTF | Iron-binding protein in breast milk; LTF receptor expressed on apical surface of enterocytes |
a DCYTB , duodenal cytochrome B; DMT1, divalent metal-ion transporter 1; FLVCR , feline leukemia virus, subgroup C, receptor; FPN1 , ferroportin 1; HCP1 , heme carrier protein 1; HEPH , hephaestin; HO , heme oxygenase; LTF , lactoferrin.
Iron movement across the enterocyte is usually considered in terms of three phases or steps ( Fig. 60.1 ): (1) iron uptake—the movement of iron from the intestinal lumen across the BBM and into the enterocyte; (2) an intracellular phase where iron is stored or utilized within the enterocyte or directed to the BLM for subsequent export; and (3) the export of iron across the BLM and into the interstitial fluids which bathe the serosal side of enterocytes, the so-called transfer step. The small intestine is able to utilize different forms of iron and it is the BBM proteins that must deal with this variety. The utilization of inorganic, or nonheme, iron (which is likely bound to low molecular weight organic acids) is particularly important and has been most extensively studied, but iron can also be delivered to enterocytes as heme, ferritin, or lactoferrin (as discussed later). Irrespective of the dietary source, it is likely that much of the iron taken up into enterocytes enters a common cytosolic “labile” iron pool and is subsequently exported via a common FPN1-mediated pathway.

Iron absorption is a rapid process. Following the administration of a radioactive dose of iron into the lumen of the proximal small intestine, radioactivity usually appears in the circulation within 15 s, and within minutes, 60%–80% of the total amount ultimately absorbed is transferred into the body. This initial phase of rapid iron uptake is followed by a slower rate of transfer that continues for 12–48 h. It is likely that this iron was initially retained within enterocytes in ferritin. Some iron stored in ferritin, however, never makes it into the circulation since it is lost when mucosal cells are exfoliated at the end of their lifespans. During iron deficiency, not only is the total amount of iron absorbed greater but also the fraction retained within the enterocyte is much smaller.
The quantity of iron absorbed by the intestine is related to the luminal iron concentration in a biphasic manner. At low iron concentrations, there is a direct relationship between the iron concentration and absorption, but with increasing amounts of luminal iron, proportionally smaller amounts of the nutrient are absorbed, that is, the process is saturable. This in turn reflects specific iron uptake processes. However, iron absorption never fully saturates, and with very high doses of iron, a linear relationship between the luminal iron concentration and the amount absorbed is again observed. These findings suggest that iron absorption is a saturable, carrier-mediated process at physiological intraluminal iron concentrations, but that a high-capacity, nonspecific process (possibly paracellular flux) is operating at higher concentrations. This same phenomenon has been described for the absorption of other essential minerals, notably calcium. Very large doses of oral iron can overcome feedback mechanisms, which normally limit iron absorption, likely reflecting the nonspecific component. The saturable process represents what we now recognize as the normal physiological DMT1/FPN1-mediated iron absorption pathway that will be described in detail below.
In the past, there was considerable debate over the issue of whether brush-border uptake or basolateral efflux was the rate-limiting step in iron absorption (summarized in Ref. ). Teleologically, it makes sense that the basolateral transfer step is rate limiting as the amount of iron crossing the BLM cannot exceed that crossing the BBM. The intestinal epithelium essentially acts as a buffer to control dietary iron assimilation. Iron can move across the BBM and into the enterocyte, but whether it proceeds any further depends on the export capacity of the BLM. Iron not absorbed immediately is stored within ferritin, but this iron can ultimately be utilized later if needed to meet metabolic demands. Although early kinetic and physiological investigations provided results that were somewhat conflicting, the consensus from these studies was that basolateral transfer was indeed rate limiting. In recent years, with improved understanding of the molecular basis of iron absorption and its regulation, strong support for the regulatory role of the basolateral transit of iron has been provided. This will be considered in greater detail below.
60.4.2
BBM Uptake of Iron
60.4.2.1
Ferric Iron Reduction
Much of the dietary iron in the intestinal lumen is in the oxidized or ferric (Fe 3 + ) form, yet it is ferrous iron (Fe 2 + ) that is more soluble and is the substrate for the major BBM iron transporter, DMT1. Ferric iron must thus first be reduced before it can be utilized. As noted above, endogenous (e.g., gastric secretions) and dietary (e.g., ascorbic acid) factors function to promote iron reduction, but enzymatic iron reduction is probably required, particularly when iron absorption is high. Several biochemical studies have provided evidence for the existence of a BBM-associated ferric reductase (or “ferrireductase”), but whether one or multiple enzymes are involved is unclear. One identified candidate ferrireductase is the transmembrane protein DCYTB. This protein has iron reductase activity in vitro and an antibody against DCYTB inhibits iron reduction in duodenal samples. Interestingly, DCYTB activity is functionally coupled to intracellular ascorbate, which may provide the electrons that ultimately reduce ferric iron. The expression of DCYTB is highest in the proximal small intestine, and expression is enhanced by stimuli that increase iron absorption, such as iron deficiency and hypoxia. However, when the gene-encoding DCYTB was deleted in mice, no overt phenotype was noted, demonstrating that DCYTB is not essential for iron absorption. Subsequent, more detailed iron absorption studies using these mice showed that they do indeed have a mild iron absorption defect. Moreover, recent investigations support the contention that DCYTB is the only iron/hypoxia-regulated ferrireductase in mice. Nevertheless, it is likely that there is redundancy in this system and that one or more additional ferrireductases exist on the BBM.
60.4.2.2
The Role of Divalent Metal-Ion Transporter 1 (DMT1)
Iron can transit the BBM in a variety of forms, but quantitatively, the transport of nonheme iron via DMT1 appears to be most important. DMT1 is expressed in most body cells where it is required for the uptake of TF-bound iron via the transferrin cycle, but it also plays a more specialized role in facilitating the uptake of iron from the intestinal lumen. When the Slc11a2 gene (encoding DMT1) was specifically deleted from the small intestine in mice, and in rodents carrying mutations in Slc11a2, severe anemia was observed, suggesting that DMT1 provides the major route for iron transport into the enterocyte.
DMT1 is an integral membrane protein with 12 predicted transmembrane domains. As its name suggests, it transports ferrous iron (Fe 2 + ) rather than ferric iron (Fe 3 + ). DMT1 has also been shown to transport other cations, including Cu, Mn, Zn, Co, and Cd. Despite this, however, the major consequence of mutation or loss of DMT1 is severe IDA in rodents and humans. Iron thus appears to be the major physiological substrate of DMT1. This point is further emphasized by the fact that mice lacking DMT1 in only the intestine have impaired intestinal iron transport and severe anemia, but copper and manganese absorption and homeostasis are unaltered. DMT1 functions as a proton-coupled cotransporter; the movement of ferrous iron into IECs is coupled to the cotransport of protons. DMT1 thus functions well at low pH, so it is ideally suited to transport iron in the acidic environment of the proximal small intestine. The cotransported protons are, at least in part, provided by the action of an apically expressed sodium‑hydrogen exchanger (NHE3). Mutatagenesis studies of DMT1 have shown that transmembrane (TM) domains 1 and 6 are critical for iron binding and uptake, as well as proton coupling. Residues in TM segments 3, 4, and 9 are also required for efficient metal ion transport, and amino acid residues in several other regions have been shown to play some role in iron transport.
The pattern of DMT1 expression is consistent with its role in iron absorption. Although it is expressed in almost all body cells (where it is involved in iron export from endosomes as part of the TF cycle), expression is particularly high in the proximal small intestine, the main site of iron absorption. At the subcellular level, DMT1 is located on the BBM when the demand for iron transport is high, but when cellular iron levels are adequate and body iron stores are replete, the protein is predominantly found in intracellular membranes. Moreover, if animals on an iron-deficient diet are transferred to a diet of higher iron content, DMT1 was rapidly internalized from the BBM and then degraded. Some proteins related to DMT1 trafficking have been recently identified mainly in hepatocytes, including Nedd4 family interacting proteins 1 and 2 (NDFIP1/2) that regulate DMT1 levels by acting as adaptors to recruit a ubiquitin protein l, thus mediating DMT1 degradation in the proteasome. Whether such a mechanism is operational in the intestine is, however, not currently known. The iron-dependent trafficking of DMT1 is likely to play a protective role upon acute exposure to high dietary or supplemental iron.
DMT1 mRNA and protein expression is strongly upregulated by iron deficiency and during other conditions where iron absorption is stimulated and reduced with iron loading and during infection and inflammation. For example, DMT1 expression is induced after Roux-en-Y gastric bypass surgery (which is commonly associated with iron depletion), and decreased DMT1 expression may contribute to the iron deficiency commonly associated with IBDs. The presence of a consensus iron-responsive element (IRE) (a stem-loop structure) in the 3′ untranslated region of the DMT1 mRNA molecule provides a mechanism for the iron-dependent regulation of DMT1 via the IRE/iron-regulatory protein (IRP) system. During iron-deficient conditions, IRPs bind to the 3′ IRE and protect the DMT1 mRNA from degradation. Upon iron deficiency, DMT1 mRNA is thus stabilized and more DMT1 protein is produced via enhanced translation. Conversely, when iron is plentiful, the IRPs do not bind to the DMT1 transcript and it is more rapidly degraded, thus decreasing protein levels and reducing iron uptake from the intestinal lumen. Interestingly, there are two 3′ splice variants of the DMT1 transcript, one with an IRE and one without, although it is the IRE-containing splice variant that is particularly abundant in the duodenum. The finding that the non-IRE variant can still be regulated by iron (increased in iron deficiency and decreased with iron loading) suggests that transcriptional regulation may also be occurring. This likely reflects transcriptional activation of the Slc11a2 gene (encoding DMT1) by a hypoxia-inducible transcription factor, HIF2α (as outlined in a subsequent section of this chapter). Furthermore, it is also possible that DMT1 is regulated posttranslationally via a hepcidin-mediated mechanism, whereby hepcidin signaling causes DMT1 to be internalized from the enterocyte BBM and targeted for degradation in the proteasome.
60.4.2.3
Heme Absorption
Heme, principally derived from myoglobin and to a lesser extent hemoglobin, is considered to be an important source of iron, particularly in diets that contain red meat. Heme is liberated from the porphoryin ring by proteases in the intestinal lumen and it is this released heme that is presented to the BBM. Heme iron is considered to be more bioavailable than nonheme iron, and because the iron is effectively bound within the porphyrin ring, heme iron absorption is largely unaffected by substances that inhibit the absorption of nonheme iron. In fact, it has been suggested that although only 10% of the iron in a typical Western diet may be in the form of heme, the heme pool contributes up to 50% of the total iron absorbed.
Despite the important contribution of heme to overall dietary iron intake, the mechanism of heme absorption remains very poorly understood. Early studies provided evidence for the presence of a specific heme-binding protein on the BBM in several species, although its identity remains unknown. Associated morphological studies suggested that after binding to the BBM, heme was internalized in endocytic vesicles, which ultimately fused with lysosomes where the heme was degraded. There is also evidence that heme might be transported directly across the BBM. Recently, a low-affinity heme transporter designated heme-carrier protein 1 (HCP1) was identified ( Fig. 60.2 ). HCP1 has some characteristics consistent with a role in heme transport in the gut, including strong expression on the BBM and increased expression in response to hypoxia, a known stimulus for iron absorption. However, subsequent studies have shown that HCP1 is also a high-affinity folate transporter (termed the proton-coupled folate transporter; PCFT). Patients with mutations in HCP1/PCFT are phenotypically folate deficient rather than iron deficient, so whether this protein contributes to heme absorption in the physiological setting is unclear. The mechanism by which dietary heme is absorbed across the BBM has thus not been clarified.

After crossing the apical membrane, heme has two potential fates: being catabolized within the enterocyte or trafficked intact across the BLM and into the circulation. The latter does not seem to be a major route, as studies which followed the fate of heme with a radioactive iron center showed that the vast majority of the heme-derived iron appears in the circulation bound to transferrin, that is, it appears to exit the cell via the same pathway as nonheme iron. Despite this, two heme export proteins have been identified that could function in the enterocyte: FLVCR (feline leukemia virus, subgroup C, receptor) and ABCG2 (also referred to as the breast cancer resistance protein). FLVCR plays a role in reducing excess heme levels in erythroid cell precursors and in heme release from macrophages. ABCG2 appears to facilitate heme efflux from immature erythroid cells. Both proteins are expressed in enterocytes and intestinal cell lines (with ABCG2 being localized to the BBM), but whether these proteins play a role in heme iron absorption or in protecting IECs against heme-related toxicity remains to be determined.
The dominant fate of heme within the enterocyte is degradation and this is mediated by heme oxygenases (HOs). The majority of studies have concluded that HO-1 is the main enzyme involved in processing newly absorbed heme, but this has not been proven definitively. A recent study showed that lack of intestinal HO-1 did not impair heme absorption in mice, although the authors suggested that mice are poor utilizers of dietary heme. Recent localization data have also implicated HO-2 in the process and have suggested that this is the more likely of the two enzymes involved in releasing iron from intracellular heme. It is possible that both enzymes contribute to heme degradation in enterocytes. Whichever the case though, after iron is released from heme, it is predicted to enter the same transit pool as nonheme iron and thus be transported across the BLM via FPN1.
Like nonheme iron, heme iron transport in the intestine is regulated by iron demand, being enhanced when iron requirements increase. However, heme iron absorption is not regulated over as wide a range as nonheme iron absorption. This seems somewhat surprising since basolateral transfer is considered rate limiting for absorption and both heme and nonheme iron appear to utilize the same efflux pathway. This apparent discrepancy can be reconciled by the finding that it is the degradation of heme that is likely rate limiting for absorption. While HO-2 shows limited regulation, HO-1 can be induced by iron deficiency, providing a mechanism for the (more limited) regulation of heme iron absorption.
60.4.2.4
Other Dietary Iron Sources—Ferritin and Lactoferrin
While it is likely that most nonheme iron is ultimately released from dietary components prior to transport by the enterocyte BBM, protein-bound forms of iron could also contribute to total iron intake. Much of the iron within plant cells is stored within ferritin, and there thus have been suggestions that ferritin could contribute to dietary iron intake. Furthermore, there has been considerable interest in the use of plant ferritins as iron supplements. A number of studies have provided evidence that ferritin iron is bioavailable in both rodents and humans. However, whether ferritin is broken down in the intestinal lumen before its iron can be utilized or if it crosses the BBM intact is not clear. A number of studies have shown that ferritin can be taken up via an endocytic process in Caco-2 cells (which have some enterocyte-like characteristics). Whether this reflects the situation in vivo has not yet been determined definitively, but a recent investigation supports this possibility. These authors suggested that dietary ferritin taken up by enterocytes functions as an exchangeable iron pool that can slowly release iron over time.
Lactoferrin is the major iron-binding protein in breast milk. It has been proposed to act as a bacteriostatic agent, to influence the immune system and to have positive effects on the growth of the intestinal epithelium. It is also a potential source of dietary iron for the infant, although mice deficient in the lactoferrin gene survive the suckling period without becoming any more iron deficient than their wild-type counterparts. Nevertheless, recombinant and bovine lactoferrin have been shown to improve iron status in human supplementation trials. How lactoferrin might exert its effects is unclear. A lactoferrin-binding protein/receptor has been identified on the enterocyte BBM, but whether lactoferrin and its iron can be utilized through this pathway has yet to be determined. More recent work has suggested that lactoferrin functions to sequester iron as part of the acute-phase response to microbial infection. This study also reported that the lactoferrin-iron complex can subsequently be sequestered by a secreted lactoferrin-binding protein, glyceraldehyde-3-phosphate dehydrogenase, and further that entire complex can then be taken up across the BLM into enterocytes.
60.4.3
The Intracellular Phase of Iron Absorption
The fate of iron after it crosses the BBM is poorly understood. Within the enterocyte, as with all cells, iron is considered to enter an exchangeable “pool,” the nature of which is not well defined. It seems likely that iron is present in the cytoplasm as chelates with small organic acids such as citrate or amino acids, or it could be bound with low affinity to intracellular proteins. Membrane permeant iron chelators such as desferrioxamine inhibit iron absorption, presumably by chelating iron in this intracellular pool. For another redox-active and potentially toxic transition metal, copper, several specific and high-affinity intracellular- binding proteins, or chaperones, have been identified ; however, with one exception (see below), intracellular iron chaperones have not been identified to date. Irrespective of what iron is bound to in the intracellular pool, it is clear that this iron can have one of three fates: (1) if body iron requirements are high, then the metal can move quickly across the BLM and into the bloodstream; (2) if body iron demand is low, the metal is stored within ferritin in the enterocyte; or (3) enterocytes may also utilize newly absorbed iron for metabolic purposes, but it is likely that most of the iron required in enterocytes is obtained when the cells are produced from stem cells in the intestinal crypts. Furthermore, the intracellular iron pool can influence posttranscriptional regulation of genes encoding proteins involved in cellular iron metabolism, as discussed below.
The best characterized pool of iron in the enterocyte is that within the iron-storage protein ferritin. The 24 subunits of apo -ferritin form a hollow sphere which can accept up to 4500 atoms of iron. Iron not required for basolateral transport and in excess of cellular metabolic needs is incorporated into ferritin, but this iron can subsequently be accessed if metabolic demand increases, at least in the short term. If the iron is not reutilized, the ferritin is lost from the body in the feces after the enterocytes are exfoliated into the intestinal lumen. When very large amounts of iron are incorporated into ferritin, ferritin complexes form and ultimately fuse with lysosomes, leading to the degradation of the protein shell; the resulting amorphous mixture of peptides and iron oxide is known as hemosiderin. The translation of ferritin mRNA is regulated by iron such that increased cellular iron levels lead to the synthesis of ferritin, which sequesters the iron and prevents it from accumulating in the cytoplasm to toxic levels. In this sense, ferritin would be expected to play a passive role in iron absorption. However, it has been shown that overexpression of ferritin in cultured cells depletes cytosolic iron, and there is evidence that a similar effect can occur in enterocytes in vivo under certain pathological circumstances. Thus, the possibility that ferritin can actively modulate the passage of iron across the enterocyte cannot be excluded. A recent study, in fact, suggests that ferritin functions in parallel with hepcidin to prevent excessive iron absorption.
Although there is limited evidence to indicate that protein chaperones might play a role in the intracellular trafficking of iron, it was recently demonstrated that poly (rC)-binding protein 1 (PCBP1) was able to facilitate iron loading onto ferritin. More recent work demonstrated that PCBP2, a PCBP1 homolog, interacts with DMT1 and FPN1, thus possibly functioning in a similar manner to copper chaperones, but whether this occurs in enterocytes has not yet been investigated.
60.4.4
BLM Transfer of Iron
The movement of iron across the BLM of the enterocyte (the transfer phase) plays a critical role in iron absorption. It is the BLM that is the main interface with the body and as such, it receives information about body iron requirements. This in turn makes it the primary site for the regulation of dietary iron intake. The membrane passage of iron is mediated by the iron export protein ferroportin 1 (FPN1), but an iron oxidase is also required for efficient transport. The iron export‑iron oxidation functional couple is considered in detail below.
60.4.4.1
Ferroportin 1
FPN1 is the only known exporter of nonheme iron in mammals. It was identified almost simultaneously by three groups, one of which was specifically seeking genes encoding proteins involved in iron absorption. FPN1 is expressed in almost all body cells, as most cells require a mechanism for divesting themselves of potentially toxic, excess iron. The protein is expressed at particularly high levels in cells that must export large quantities of iron as part of their normal daily activities, including enterocytes, macrophages of the RE system (including hepatic Kupffer cells), and hepatocytes.
FPN1 is an integral membrane protein with 12 predicted transmembrane domains. Like DMT1, it uses ferrous iron as a substrate, but there is limited information on the mechanism by which the protein transports iron. The analysis of patients with mutations in the FPN1 gene ( SLC40A1 ) has led to the identification of a number of residues involved in iron transport, but detailed structure-function studies have yet to be carried out. Human FPN1, when heterologously expressed in Xenopus oocytes, transports Fe, Zn, and Co, but not Cu, Cd, or Mn; however, complementary in vivo studies by these investigators in mice suggested that iron is the most important substrate. In contrast, a recent study in mice expressing a mutant form of FPN1 (called flatiron mice) suggested that lack of fully functional FPN1 impaired Mn metabolism. Furthermore, it is unclear whether FPN1 functions as a monomer or a dimer. Genetic evidence suggests that a dimer is more likely, although recent evidence has shown that purified, detergent-solubilized FPN1 behaves as a monomer. FPN1 is strongly expressed in the proximal small intestine, the major site of iron absorption, but its expression is much lower in the distal parts of the small intestine and the colon. Within the intestinal epithelium, expression is restricted to the mature absorptive enterocytes, and immunohistochemical studies have shown that the protein is found predominantly on the BLM, a localization highly consistent with a role in mediating iron efflux from IECs.
The importance of FPN1 in iron homeostasis has been demonstrated by deletion of the gene in mice and zebrafish, and by studies in humans carrying mutations in the gene encoding FPN1. Global knockout of FPN1 in mice is embryonic lethal attesting to its necessity in normal development. Intestine-specific knockout of the gene leads to a severe IDA, demonstrating that FPN1 is required for normal iron absorption. Zebrafish lacking FPN1 also have a severe IDA and show strong iron accumulation in macrophages in the liver and spleen. It is more difficult to assess iron absorption directly in this species, but the data obtained were consistent with reduced dietary iron intake. Mutations in SLC40A1 (encoding FPN1) in humans are uncommon, but they still represent an important class of human iron-loading disorders. There are two basic clinical presentations depending on the nature of the mutation in SLC40A1 . Mutations affecting the localization and/or iron transport capacity of the protein lead to impaired iron absorption and iron accumulation in macrophages, confirming the importance of this protein in iron delivery to the plasma. However, some patients have mutations that alter the capacity of FPN1 to bind to the iron-regulatory hormone hepcidin. In this case, iron absorption is increased as the usual feedback mechanism limiting iron uptake is disrupted. Such studies highlight the important role FPN1 plays in the regulation of iron absorption, as considered in more detail below.
The regulation of FPN1 appears relatively complex. Early studies showed that FPN1 expression in the duodenum was inversely related to body iron load, which is a seemingly appropriate physiological response. In other tissues, however, such as the liver, FPN1 expression positively correlates with body iron status; that is, FPN1 expression is low during iron deficiency and high when body iron levels are elevated. This response could reflect a cellular mechanism to prevent iron depletion and iron toxicity. An explanation for this regulation is provided by the demonstration that the FPN1 mRNA contains a 5′ IRE. Thus, like the ferritin transcript, when iron levels are high, the IRE will not be occupied by an IRP and translation will proceed, and conversely, when intracellular iron is low, an IRP will bind to the FPN1 IRE and block translation. However, this does not explain the opposite pattern of regulation in the intestine. The resolution of this problem has come from the demonstration that there are two FPN1 transcripts, one which contains a 5′ IRE and one which does not. Under iron-deficient conditions in the intestine, translation from the IRE-containing splice variant is diminished, but expression of the non-IRE variant is increased, thereby enabling absorption to proceed. This increase in the expression of the non-IRE FPN1 transcript could be mediated directly by iron (or lack of iron), or it could reflect transcriptional induction by HIF2α in response to hypoxia and/or low iron. Early studies indeed showed that hypoxia was able to stimulate the expression of FPN1. Transcriptional and translational regulation of FPN1 expression helps to set levels of the protein in enterocytes, but superimposed upon this is the hepcidin- mediated removal of FPN1 from the plasma membrane. The latter is the critical factor in enabling iron absorption to respond to alterations in iron requirements, particularly in the setting of high body iron levels, which will be considered in more detail below.
60.4.4.2
The FOXs Hephaestin and Ceruloplasmin
Iron traverses the BLM through FPN1 in the ferrous form but iron oxidation to the ferric form is required for binding to transferrin, the dominant iron-transport protein in the plasma. Iron efflux from the enterocyte thus requires the coordinated actions of both an iron exporter and a FOX. In the small intestine, one such FOX is a protein known as hephaestin (HEPH), which was identified by positional cloning as the gene responsible for the phenotype of the sex-linked anemia ( sla ) mouse. These mice have moderate IDA in early life and early kinetic studies showed that it was the basolateral transfer step of iron absorption that was defective. Moreover, recently, an intestine-specific HEPH ( Heph int ) KO mouse was developed, and also a whole-body HEPH KO mouse. The latter mouse displayed a moderate impairment in intestinal iron transport, while adult male Heph int KO mice showed iron accumulation in duodenal enterocytes and mild iron deficiency. Interestingly, in all these mouse models of HEPH mutation or KO, growing, young mice are iron deficient and anemic, yet the anemia mostly resolves as mice age, suggesting that other FOXs may exist which can complement HEPH activity. FOX activity was indeed detected in cytosolic and membrane fractions of duodenal enterocytes of HEPH KO mice, perhaps revealing such alternative FOXs.
HEPH is strongly expressed in mature enterocytes of the small intestine, as would be expected of a molecule involved in iron absorption, and at relatively lower levels in several other tissues. Surprisingly, it is expressed at high levels throughout the small intestine and into the colon, whereas other important components of the intestinal iron transport machinery (e.g., DMT1, FPN1) are preferentially expressed in the proximal small intestine where most iron absorption occurs. HEPH has high amino acid sequence homology to the circulating blood protein ceruloplasmin (CP) ; however, unlike CP, it has a single C-terminal transmembrane domain. HEPH and CP both bind copper (which is incorporated biosynthetically) and can oxidize ferrous iron to ferric iron. Copper is required for enzymatic activity of both proteins, probably explaining why copper-deficient animals have defective iron absorption.
Since FPN1 mediates iron efflux from enterocytes, it is a logical postulate that it interacts with HEPH. Such an interaction has not been unequivocally demonstrated in vivo, but colocalization studies in the intestine and in intestinal cell lines suggest that this is indeed a possibility. How HEPH interacts with FPN1 to facilitate iron release from cells is nonetheless poorly understood. A recent study in glioma cells and mouse bone marrow-derived macrophages found that the FOX activity of a membrane-anchored form of CP (GPI-CP) was required for the release of iron from FPN1. In the absence of the FOX, ferrous iron remained bound to FPN1 which was then ubiquitinated, internalized, and degraded. Thus, iron oxidation stabilized FPN1 on the plasma membrane. Such a mechanism could also explain how HEPH promotes iron absorption in the intestine, but this postulate has not been tested to date.
Since CP is a secreted protein and it has been shown to be involved in facilitating iron release from a variety of tissues, it might be expected to contribute to iron absorption as it is (presumably) present in the enteric circulation. Cp-knockout mice, however, do not have an obvious defect in iron absorption. Nevertheless, more recent studies have shown that CP can contribute to iron absorption when body iron requirements are increased. For example, when erythropoiesis was stimulated in Cp-knockout mice by phlebotomy, absorption could not be increased to the same extent as it could in wild-type mice. The possibility that Cp contributes to intestinal iron absorption suggests some redundancy in the oxidative mechanism and could explain why disruption of HEPH (such as in the sla mouse) does not lead to a very severe anemia. Although CP is likely able to contribute to iron absorption, it does not appear to do as efficiently as HEPH. The reasons for this are unknown, but it may relate to the precise site where the iron oxidation takes place. Early studies showed that, at the subcellular level, most HEPH is located in an unidentified intracellular compartment, although evidence of BLM localization has been obtained more recently. It is thus conceivable that FPN1 and HEPH interact intracellularly within enterocytes. This location could be less accessible (or inaccessible) to CP, which could explain its lower efficiency in promoting iron absorption.
60.4.4.3
Iron Transit Into the Portal Blood Circulation
After iron crosses the BLM, it must make its way to the circulation, but precisely how this occurs is unclear. Newly absorbed iron is able to bind to apo -transferrin (TF), but the transcapillary exchange rate of transferrin is insufficient to account for all the iron absorbed. Furthermore, if transferrin directly received iron from the basolateral iron transport complex then apo -transferrin might be expected to bind to the BLM, but there is no compelling evidence that this is the case. For example, a recent study failed to identify any interaction between TF and HEPH. We also know that TF is not essential for iron absorption as hypotransferrinemic animals, which have no or negligible circulating TF, do not have impaired iron absorption. It thus seems likely that at least some newly absorbed iron passes into the circulation either as a low molecular weight chelate or bound with low affinity to plasma proteins such as albumin, and that it subsequently makes its way to the circulation where it is picked up by transferrin.
60.5
Regulation of Iron Absorption
Iron absorption is a highly regulated process. In times of high body iron demand absorption is increased, but when iron requirements are low, absorption is reduced. It was recognized many years ago that humans do not possess a mechanism for the active excretion of iron, so the amount of iron in the body must be determined through the regulation of iron absorption. It has now been established that the same factors that regulate intestinal iron absorption also regulate iron donation to the plasma from other sources, notably macrophages of the RE system, which phagocytize senescent red blood cells, and other iron storage sites such as hepatocytes of the liver. Furthermore, as will be discussed below, physiological signals reflecting body iron demand act through the same regulatory pathway to modulate iron flux rates into the serum from each of these sources. The most important driving force for this regulation is iron supply to the erythroid marrow to support hemoglobin synthesis, as this represents the major site of iron utilization in the body. All nonerythroid cells also require iron for normal metabolic functions, so overall body iron levels are also an important regulator of iron absorption.
60.5.1
Stimuli That Alter Iron Absorption
Changes in the two largest pools of iron within the body—storage iron and iron in the erythroid mass—exert the greatest influence on iron absorption. Absorption is increased when body iron stores are low or when the erythropoietic rate is increased, and vice versa. Absorption is also increased during chronic hypoxia, pregnancy and the suckling period (as discussed in detail below). The discussion here will focus largely on nonheme iron absorption. The absorption of heme iron is also affected by variations in iron status and erythropoiesis, but the magnitude of change is smaller. Moreover, after heme has entered the enterocyte and iron has been released from the protoporphyrin ring, the liberated iron is predicted to follow the same pathway into the body as recently absorbed nonheme iron, and thus, this component of intestinal iron transit should be regulated similarly for both dietary sources of iron.
The level of body iron stores is a major factor regulating nonheme iron absorption in a normal individual. In both humans and in animals, an inverse relationship exists between iron stores and absorption, with depleted stores leading to increased absorption and elevated stores reducing it. Finch coined the term the “store regulator” to describe the regulation of iron absorption by body iron levels. The body appears to have an optimum level of storage iron and in a normal adult male human this is approximately 1 g. If stores drop below this level, absorption is increased until they are replenished, whereas if stores are elevated, absorption will decline until equilibrium is regained. Indeed, under normal conditions, once iron stores have reached the optimum level, it is difficult to induce the body to accumulate more even if the iron content of the diet is increased significantly.
The other major physiological process influencing iron absorption is erythropoiesis. The largest pool of iron in the body is in the hemoglobin of circulating erythrocytes, and consequently the erythroid marrow is the single most significant sink for iron. The rate of erythropoiesis has been shown to have a direct relationship with iron absorption in animal studies. In humans, iron absorption is also enhanced when erythropoiesis is stimulated by bleeding or acute hemolysis or following androgen or erythropoietin treatment, and decreased following hypertransfusion or radiation treatment. The capacity of the body to increase iron absorption in response to an increased erythroid iron demand is very high, and in IDA, for example, individuals may absorb over 20 mg/day providing dietary iron supply is sufficient. The magnitude of this response is far greater than that typically seen with the store regulator, and thus the term “erythroid regulator” has been proposed. As will become apparent below, experimental advances have blurred the distinction between the store and erythroid regulators. The erythropoietic effect on absorption is very strong and under many circumstances it can override the effect of changes in body iron stores. In β-thalassemia for example, high iron absorption continues in the setting of elevated body iron stores due to the high marrow demand for iron, and in the iron-loading disorder hereditary hemochromatosis (HH), venesection therapy will stimulate absorption even though iron stores may be greatly elevated. Nevertheless, these regulatory factors each exert their effects and, for example, the high drive to increase iron absorption in β-thalassemia can be blunted when body iron levels become very high.
The adequacy of iron supply to the marrow and the rate of effective erythropoiesis are important determinants of iron absorption. However, an increased rate of erythropoiesis per se need not stimulate iron absorption. For example, in chronic hemolytic states, the absorption rate is usually normal despite increased erythroid activity. In this situation, as long as the return of iron to the plasma from the destruction of senescent red cells or from storage is sufficient to provide for the increased erythroid activity, absorption will not be increased. Another example is found in a murine model of mild β-thalassemia where erythroid iron demand is increased, but iron absorption is not. However, once the ability to meet the marrow’s requirements by catabolism of red cells or mobilization of storage iron is exceeded, an increase in iron absorption will result. When erythroid demand is very high and erythropoiesis is markedly ineffective (for example, in iron loading anemias such as β-thalassemia and sideroblastic anemia), internal iron traffic cannot meet the marrow demand and iron absorption is elevated.
Iron absorption is also known to be increased by a number of other stimuli that are worth mentioning briefly. Absorption is increased under hypoxic conditions and returns to normal after the hypoxic stimulus is removed. Part of the effect of low oxygen tensions can be ascribed to alterations in the rate of erythropoiesis, but a component of the response is independent of these effects. For example, early studies showed that an effect of hypoxia on iron absorption can be observed before a response from the erythroid marrow, suggesting that hypoxia can exert direct effects on the intestinal enterocyte. Studies in recent years have provided a mechanism for this by describing effects of hypoxia on both the body iron homeostatic machinery in the liver and on the expression of genes encoding iron transport proteins in the intestine. Absorption can also be increased in the perinatal period. Moreover, during pregnancy, there is a high demand for iron to provide for an expansion of the maternal erythroid mass and the iron requirements of the developing fetus. Iron absorption is increased several fold to meet these requirements, but it seems likely that the drive for this increase is provided by reduced maternal iron stores and hypoxia rather than by a pregnancy-specific factor. Finally, during the suckling period, mammals absorb iron from breast milk extremely efficiently. Iron demands are certainly very high at this time due to rapid growth and no doubt provide a strong driving force for absorption, but the immature intestine has some quite different properties from the adult intestine so the passage of iron across the intestinal epithelium during suckling may use pathways that are not available in the adult. Each of these physiologic situations that increase iron absorption will be considered in more detail below.
60.5.2
Hepcidin and the Regulation of Body Iron Homeostasis
The liver-derived, peptide hormone hepcidin is now considered the main systemic regulator of body iron homeostasis, including intestinal iron absorption, particularly in the setting of increased body iron stores and during infection and inflammation. Hepcidin was originally identified in plasma and urine as an antimicrobial peptide and was subsequently linked to iron homeostasis by two groups. In one of these investigations, inactivation of the Usf2 gene in mice inadvertently disrupted expression of the adjacent Hamp1 (hepcidin) gene and the mice subsequently developed significant iron overload. In the other study, the Hamp1 gene was identified as being strongly upregulated in the liver of mice that had been loaded with iron. These studies showed that the expression of hepcidin was inversely related to body iron intake and implied that hepcidin was a repressor of iron absorption. This was confirmed when it was demonstrated that mice engineered to overexpress hepcidin developed a severe IDA. Other studies also showed that there was a close inverse relationship between the expression of hepcidin by the liver and the expression of iron transporters in the duodenum. The importance of hepcidin in maintaining body iron balance is well exemplified by patients with mutations in the HAMP gene. Such patients develop an early onset, severe iron-loading disorder (juvenile hemochromatosis) as a result of an uncontrolled high level of intestinal iron absorption. Iron absorption is increased when hepcidin levels are reduced and disturbances in hepcidin production underly many inherited iron-loading diseases, as will be discussed below.
The major site of hepcidin synthesis is hepatocytes in the liver. Most circulating hepcidin is likely derived from these cells, but some studies have shown that small amounts of hepcidin can be produced locally in other tissues, including, but not limited to, macrophages and adipocytes. Of particular interest in the context of iron absorption is the demonstration that hepcidin is expressed in gastric parietal cells, where it may play a role in acid secretion, which promotes iron absorption in the duodenum. Hepcidin is produced as a preprotein of 85 amino acids, and it is subsequently processed by furin-mediated cleavage and secreted from the cell. In the plasma, hepcidin peptides of 20, 22, and 25 amino acids have been detected, but it is the 25 amino acid form that is responsible for the bulk of its biological activity.
Hepcidin exerts its profound effects on body iron metabolism by acting on FPN1 to reduce iron export from cells. While this activity has only been shown directly for a few cell types, it is likely that hepcidin acts on most body cells in this way. Duodenal enterocytes are one of the major targets of hepcidin and this explains its effects on iron absorption. Other important targets include RE macrophages and hepatocytes. Hepcidin interacts with FPN1 on the plasma membrane and facilitates the internalization and degradation of FPN1, thus reducing iron efflux. Although full details have not been elucidated, the ubiquitination of multiple cytoplasmic lysines is required for the internalization of FPN1 following hepcidin binding. Early reports suggesting that hepcidin binding leads to phosphorylation of tyrosine residues on FPN1 by Jak2 kinase have not been independently substantiated. After internalization, the protein is targeted for lysosomal degradation. Since FPN1 is expressed on the enterocyte BLM, the action of hepcidin provides a molecular explanation for why the basolateral transfer step is rate limiting for iron absorption and why hepcidin levels are inversely related to iron absorption.
60.5.3
The Regulation of Hepcidin
Consistent with its role as the central regulator of body iron traffic, hepcidin synthesis is decreased when iron demands are high, for example, during iron deficiency, when erythropoiesis is stimulated and in pregnancy, leading to increased iron absorption and release of storage iron. Conversely, hepcidin production is increased when the body is iron replete, resulting in reduced intestinal absorption and the storage of excess iron in RE macrophages and hepatocytes. Hepcidin expression is also stimulated by infection and inflammation, resulting in the hypoferremia associated with the anemia of chronic inflammation.
Changes in the level of circulating hepcidin likely explain variations in iron absorption, but an important question relates to how hepcidin production is coordinated with body iron status. Important clues to how this occurs have come from the analysis of inherited iron-loading disorders, collectively known as hereditary hemochromatosis (HH). In a normal individual with an increased body iron load, iron absorption is reduced to prevent excessive tissue iron accumulation; however, in hemochromatosis patients, this does not occur and iron absorption is inappropriately increased in relation to metabolic needs. Mutations in the HAMP gene lead to an early-onset, severe form of iron loading known as juvenile hemochromatosis. Juvenile hemochromatosis can also be caused by mutations in the hemojuvelin ( HJV ) gene. However, the most common form of inherited iron loading has a far milder phenotypic presentation and an adult-onset, which results from mutations in the HFE gene. On average, approximately 1 in 200 individuals in populations of northern European origin are homozygous for the major HFE mutation (C282Y) that increases the risk for developing pathologic iron overload. A rarer form of adult-onset hemochromatosis results from mutations in the transferrin receptor 2 ( TFR2 ) gene, but the presentation of these patients, both clinically and histologically, is very similar to patients with HFE-associated hemochromatosis. Despite variations in the severity of these four forms of HH, they share several key features: (1) iron absorption is increased in each case; (2) iron is deposited in the liver in a periportal distribution and predominantly in hepatocytes; (3) RE macrophages are relatively iron deficient (particularly in the early stages of the diseases); and (4) the mutated gene in each case is strongly expressed in hepatocytes. These commonalities suggested that these genes encode proteins that function in the same regulatory pathway ( Fig. 60.3 ). Consistent with this interpretation, subsequent studies showed that all of these diseases share the critical feature of inappropriately low or absent hepcidin expression in the setting of increased body iron levels.

Analysis of the role played by HJV in hepcidin regulation has proved particularly rewarding. HJV is one of a family of proteins known as repulsive guidance molecules (RGMs). Other members of this family, unlike HJV, are involved in development of the central nervous system, and like HJV, have been shown to act as cofactors for the signaling of the bone morphogenetic proteins (BMPs). HJV is a BMP coreceptor and signals through this pathway to increase hepcidin expression. The BMPs are members of the TGF-β superfamily of ligands and act through cell-surface BMP receptors to activate the multifunctional SMAD signaling pathway. Mice in which the key signaling component SMAD4 is deleted develop severe iron overload, indicating the importance of this pathway in iron homeostasis. A series of subsequent studies has shown that BMP/SMAD signaling is critical for HAMP expression and that many of the factors that influence hepcidin levels do so through their effects on this pathway. A range of BMPs have been shown to stimulate hepcidin gene transcription, but it appears that BMP6 is likely the most important isoform in vivo . BMP6 is a strong stimulator of hepcidin expression and Bmp6-deficient mice have reduced hepcidin expression and display body iron loading. Furthermore, BMP6 expression positively correlates with body iron levels, providing evidence that BMP6 could be an important link between body iron requirements and hepcidin expression. Interestingly, BMP6 appears to be expressed most strongly in nonparenchymal cells of the liver, implying that it might exert its effects on hepcidin expression in a paracrine manner.
HJV exists in both a membrane-bound and a soluble form (sHJV). While the membrane-bound form acts as a BMP coreceptor to stimulate hepcidin expression, sHJV has been shown to antagonize signaling via the BMP-SMAD pathway, presumably by competitively inhibiting the binding of membrane-bound HJV to BMPs. The release of sHJV requires the protease furin and furin levels are increased by iron depletion and hypoxia, providing another potential link between body iron status and hepcidin expression. Two other proteins have been shown to play important roles in modulating the signaling of HJV through the BMP/SMAD pathway. The first is a membrane protein called neogenin that appears to stabilize membrane-bound HJV and inhibit sHJV secretion. The demonstration that neogenin null mice have low hepcidin levels and develop iron overload is consistent with this. The other protein is a membrane-bound serine protease known as matriptase-2 (or TMPRSS6). The physiological substrate of matriptase-2 appears to be membrane-associated HJV and neogenin may be required for HJV cleavage by TMPRSS6. After HJV is cleaved, it can no longer act as a BMP coreceptor and hepcidin levels are reduced. This implies that the normal function of TMPRSS6 is to repress hepcidin production via the BMP-SMAD pathway.
It is well established that patients with HFE-related hemochromatosis absorb too much dietary iron relative to body iron load, and more recent studies have shown that hepcidin levels are low in such patients. However, how HFE acts to modulate hepcidin expression remains poorly understood. HFE may exert its effects on BMP/SMAD signaling, either directly or indirectly through the MAPK/ERK pathway. More recently, HFE has been shown to bind to the BMP receptor complex. Patients with HFE mutations are not as severely affected as those with HJV or HAMP mutations, indicating that HFE is an important, but not essential, part of the hepcidin regulatory pathway. This could have been predicted from earlier physiological data that showed that iron absorption was effectively regulated in hemochromatotic patients (likely carrying HFE mutations), but that at any given body iron load, iron absorption was higher in iron-loading patients than in normal controls. HFE thus appears to affect the sensitivity of the hepcidin-mediated absorption response to changes in body iron levels. A similar role can be ascribed to TFR2. Patients with mutations in TFR2 also have a relatively mild form of hemochromatosis, with a phenotype similar to subjects with HFE mutations, and reduced, but not absent, hepcidin expression. Again, how TFR2 influences hepcidin expression is not known, but MAPK/ERK signaling has been implicated. Interestingly, mice lacking both HFE and TFR2 have lower hepcidin levels and a more severe iron-loading phenotype that in animals with either deletion alone, suggesting that HFE and TFR2 exert independent effects on hepcidin expression.
Although BMP6 and furin are regulated by body iron levels and provide potential mechanisms by which body iron requirements might be relayed to the HAMP gene, it has also been postulated that HFE and TFR2 are involved in this iron-sensing process. The basis of this prediction is that both proteins have strong links to circulating diferric transferrin, which delivers iron to most cells. TFR2 binds to and is stabilized by circulating diferric transferrin, and HFE has been shown to interact with TFR1, which also binds diferric transferrin. The level of circulating diferric transferrin (or relative transferrin saturation) is thus thought to be an important signal for modulating hepcidin expression. Diferric transferrin levels increase with iron loading and decrease with iron deficiency, and a positive correlation between the concentration of diferric TF and hepcidin expression has been demonstrated. A working model has been proposed in hepatocytes whereby diferric TF displaces HFE from the HFE/TFR1 complex, leaving HFE free to interact with TFR2 and initiate signaling through the BMP/SMAD pathway or perhaps via some other mechanism. This is an attractive hypothesis, but it does not fit well with a number of in vivo studies. Despite considerable work in this area, much of it using proteins overexpressed in cell lines, we still do not have a clear picture of how the iron-sensing mechanism functions, particularly in vivo, nor indeed whether or not diferric TF provides the key regulatory signal.
Enhanced erythropoiesis stimulates iron absorption and suppresses hepatic hepcidin expression. There are several potential mechanisms for the suppression of HAMP by erythropoiesis, including iron-related downregulation of hepcidin expression in response to heightened iron demand, or tissue hypoxia as a result of reduced levels of circulating hemoglobin. Indeed, the HAMP gene contains two conserved hypoxia-response elements and it has been demonstrated that HIF1α signaling reduces HAMP transcription. While hypoxia-signaling factors may exert some influence on the body’s response (i.e., the hepcidin response) to increased erythropoiesis, there has long been evidence that additional, erythroid-specific, regulatory factors play a more significant role. Some experimental evidence has suggested that growth differentiation factor 15 (GDF15) and twisted gastrulation 1 (TWSG1) could play this role as their expression is increased when erythropoiesis was stimulated and both have been shown to act on hepatic cells to reduce hepcidin expression. Other studies, however, have not found a correlation between GDF15 and hepcidin levels. For example, hepcidin expression was appropriately regulated in response to phlebotomy in Gdf15 -knockout mice, demonstrating that GDF15 is not required for the downregulation of hepcidin expression when erythropoietic demand increases. More recently, another factor, erythroferrone, has emerged as the most likely erythroid regulator and this has now been supported by multiple studies. Precisely how erythroferrone influences hepcidin production is not known, and it remains possible that the hepcidin response to stimulated erythropoiesis involves other, as yet unidentified, regulatory factors.
A final comment should be made on the relationship between inflammation and iron absorption. In response to an inflammatory stimulus, either acute or chronic, there is a reduction in iron absorption and in the donation of iron to the plasma from RE macrophages and other iron storage sites. This is associated with a sequestering of iron within tissues. Inflammation is a strong inducer of hepcidin and the hypoferremia, and ultimately anemia, that accompanies inflammation can be explained by increased plasma hepcidin levels, although the situation is likely to be multifactorial with factors other than hepcidin contributing. Inflammation stimulates the synthesis of a range of proinflammatory cytokines including interleukin-1 (IL-1), IL-6, IL-22, and interferon-α, and it is these signaling molecules that enhance hepcidin expression. IL-6-mediated enhancement of HAMP transcription is mediated by signaling through the JAK-STAT pathway. There is a crosstalk between this pathway and the BMP-SMAD signaling pathway, as a functional SMAD-binding site in the HAMP promoter is necessary for the IL-6 stimulation of hepcidin expression. However, since hepcidin responds strongly to inflammation in Bmp6 -null mice, the necessary SMAD signal must be being supplied by a BMP receptor ligand other than BMP6.
60.5.4
Regulation at the Level of the Mucosa
Iron homeostasis is systemically regulated by the liver-derived, peptide hormone hepcidin, as outlined above, and this hormonal control is important to prevent iron overload, and in the response to infection and inflammation as part of the acute-phase response. There is, however, additional control at the level of the intestinal epithelium, and it is ultimately this epithelium that determines how much iron enters the body. These regulatory mechanisms involve local alterations in the expression of key iron transport molecules, as well as morphological changes in the epithelium, all in response to changes in iron status.
60.5.4.1
Hypoxia
The intestinal epithelium is naturally hypoxic, but more dramatically so between meals when blood flow to the gut decreases. Hypoxia also ensues when body iron levels fall below a critical threshold due to decreased hemoglobin levels. It is thus a logical postulate that the hypoxic response in the gut, which occurs during moderate to severe iron deficiency, is an important driving force mediating compensatory changes in the intestinal epithelium. At the molecular level, the hypoxic response is well primed to respond to changes in iron status as the activity of the enzyme prolyl hydroxylase (PHD), a critical part of the response, requires iron for enzymatic activity. PHD hydroxylates hypoxia-inducible transcription factors (HIFs) under normoxic conditions, thus targeting them for degradation. Earlier studies indeed revealed an increase in iron absorption during hypoxic conditions in rats and mice. Furthermore, membrane vesicle uptake studies demonstrated enhanced uptake of Fe 3 + , but not Fe 2 + , in BBM vesicles isolated from hypoxic mouse duodenum (relative to normoxic mice). When these studies were carried out in the mid-1980s, the molecular players in intestinal iron transport were unknown. More recent studies have demonstrated that hypoxia-inducible factor HIF2α is expressed at higher levels in enterocytes during iron deprivation, while HIF1α levels are unchanged. The HIFs mediate the transcriptional response to hypoxia and are regulated at the level of protein stability. During hypoxic or iron-deficient conditions, decreased PHD activity results in the stabilization of the HIFα subunits enabling them to interact with a binding partner, ARNT (or HIF1β), and subsequently the complex translocates to the nucleus where it binds to DNA and regulates gene transcription. The hypoxic response is driven by HIF1α in many cell types, but in the intestine, hypoxia preferentially stabilizes HIF2α. Multiple studies have now shown that HIF2α plays a critical role in intestinal iron absorption. The increased iron absorption that occurs in response to iron deficiency, stimulated erythropoiesis, and inherited disorders of dysregulated hepcidin production has been shown to be dependent on intestinal HIF2α. DMT1, DCYTB, and FPN1 have been shown to be direct HIF2α targets, resulting in their transcriptional induction during iron deficiency. The preferential stabilization of HIF2α has been modeled in iron-deprived Caco-2 cells, and it was suggested that many genes differentially expressed during iron deprivation are direct HIF2α targets. Future studies may indeed reveal additional HIF2α targets that are induced during iron deficiency, some of which may play novel roles in intestinal iron homeostasis. The response mediated by HIF2α, either via hypoxia or iron deficiency, may allow the intestine to rapidly respond to variations in iron demand before the systemic regulatory machinery (mediated by hepcidin) is activated.
60.5.4.2
Morphological Adaptations
In addition to alterations in gene expression, at least in-part mediated by HIF2α, there are morphological adaptations that occur in the intestinal epithelium during iron deficiency. Studies over 15 years ago demonstrated that, during iron deficiency, the zone of iron absorption extends to enterocytes closer to the crypts and also that structural adaptations occurred, including increased villus width and length. Further studies in iron-deprived rats revealed increases in villus height, mucosal thickness, and epithelial surface area in the jejunal epithelium. Consistent with this, it has been shown that iron deficiency in rats leads to an increase in the absorptive surface area of the duodenum, and that there is an increase in crypt cell proliferation (as indicated by larger numbers of mitotic figures in the crypt epithelium). Interestingly, earlier studies in iron-deficient piglets showed an opposite trend in which frank villous atrophy was evident histologically. Morphometric analysis of small intestinal specimens further showed a significant decrease in villus length, villus-to-crypt ratio, and mucosal complexity among iron-deficient piglets, while crypt depth was not altered. These studies were, however, complicated by the fact that these observations were made in piglets that had diarrhea. Whether similar compensatory adaptations occur in iron-deficient human infants or adults is not currently known.
60.5.4.3
Iron and the Mucosal Block
As iron is toxic when it rises above normal levels, the intestine has developed specific mechanisms to decrease intestinal uptake upon exposure to high iron levels, a process that has been coined the “mucosal block.” First proposed by Hahn et al . , “mucosal block” describes diminished avidity of the intestinal mucosa for iron following an orally administered blocking dose. Early studies on iron absorption indicated that such a phenomenon occurred, and subsequent studies in humans used a small-dose iron tolerance test in mildly iron-deficient healthy adults. Both 60 and 30 mg blocking doses of iron resulted in diminished absorption of a 10 mg test dose. This refractory state of the intestine lasted up to 24 h. More recent studies have sought to determine the underlying molecular mechanism responsible for this phenomenon. It was demonstrated that the DMT1 protein was acutely trafficked away from the apical membrane of enterocytes after exposure to a bolus of iron-containing food. Further studies showed that an oral iron dose led to a rapid decrease in the expression of the transcripts encoding DMT1 and DCYTB, and suggested that the mucosal block could also be due to decreased expression of the brush-border iron transport machinery.
60.6
Developmental Changes in Iron Absorption
60.6.1
Pregnancy
Iron needs increase during pregnancy and many studies have shown enhanced absorption of dietary iron during gestation. Studies in pregnant humans using either radioactive or stable iron isotopes have shown incremental increases in iron absorption from the first through the third trimesters of gestation. These studies have all suggested that iron absorption increases as the iron demands of the fetus increase. Associated with this increase is a decline in the expression of hepcidin, and this is most likely driven by the reduction in maternal body iron stores. However, not all studies have found correlations between iron and hepcidin levels during gestation, and other factors including steroid hormones associated with pregnancy, could also be involved.
A range of studies on intestinal iron transport during pregnancy have also been performed in laboratory rodents. These studies broadly parallel the investigations in humans and show increases in absorption as pregnancy progresses. In addition to increased absorption, one study in rats documented increased duodenal circumference and villus dimensions during pregnancy. Consistent with an increase in iron absorption, some studies have also documented increased expression of genes encoding iron transport proteins in the rat intestine during pregnancy.
60.6.2
Suckling
Iron homeostasis in neonatal mammals is fundamentally different from iron homeostasis in later life. The capacity of suckling infants to absorb iron is extremely high, as this is a period of very rapid growth with particularly high iron demands. Once mammals wean, however, absorption declines to adult levels. The mechanisms underlying the very high iron absorption during suckling are incompletely understood. At this developmental stage, the small intestine is relatively immature and tight junctions between epithelial cells are not fully developed, raising the possibility that the gut mucosa is “leaky” and that iron may be taken up by nonspecific processes. While this has been proposed as a possible mechanism to explain high neonatal iron absorption, it appears unlikely to be a major contributor as absorption at this stage appears to be specific and most prominent in the proximal small intestine, whereas nonspecific uptake is largely observed in more distal gut segments. More recent studies in mice have revealed that the vast majority of iron absorption during suckling is FPN1 dependent, confirming that it is a specific process. Interestingly, however, during suckling, iron absorption cannot be repressed by an increased level of systemic hepcidin, and this has been proposed to be due to a modification of FPN1, which prevents hepcidin binding. Earlier studies in humans and rodents suggested that there was little homeostatic regulation of iron transport during early infancy, but that regulation began around the time of weaning. Whether the high, unregulated absorption of iron during infancy has evolved to maximize iron intake, or whether it plays some other role, such as removing iron from the intestinal lumen to limit the growth of certain bacteria, remains to be determined. The latter is a possibility as it is generally acknowledged that full-term human infants and rodent pups are born with sufficient iron stores to take them through early postnatal life. One other particularly interesting aspect of iron absorption in early postnatal life is that the role of DMT1 in iron transport from the intestinal lumen into enterocytes appears to be unclear. One study has suggested that DMT1 is not required at this developmental stage in rats, implying that an alternative BBM transporter must be operating. Conversely, a more recent study in mice has presented evidence that DMT1 is involved. Additional studies will thus be required to resolve this apparent discrepancy and further to elucidate the functional role of DMT1 during suckling in humans.
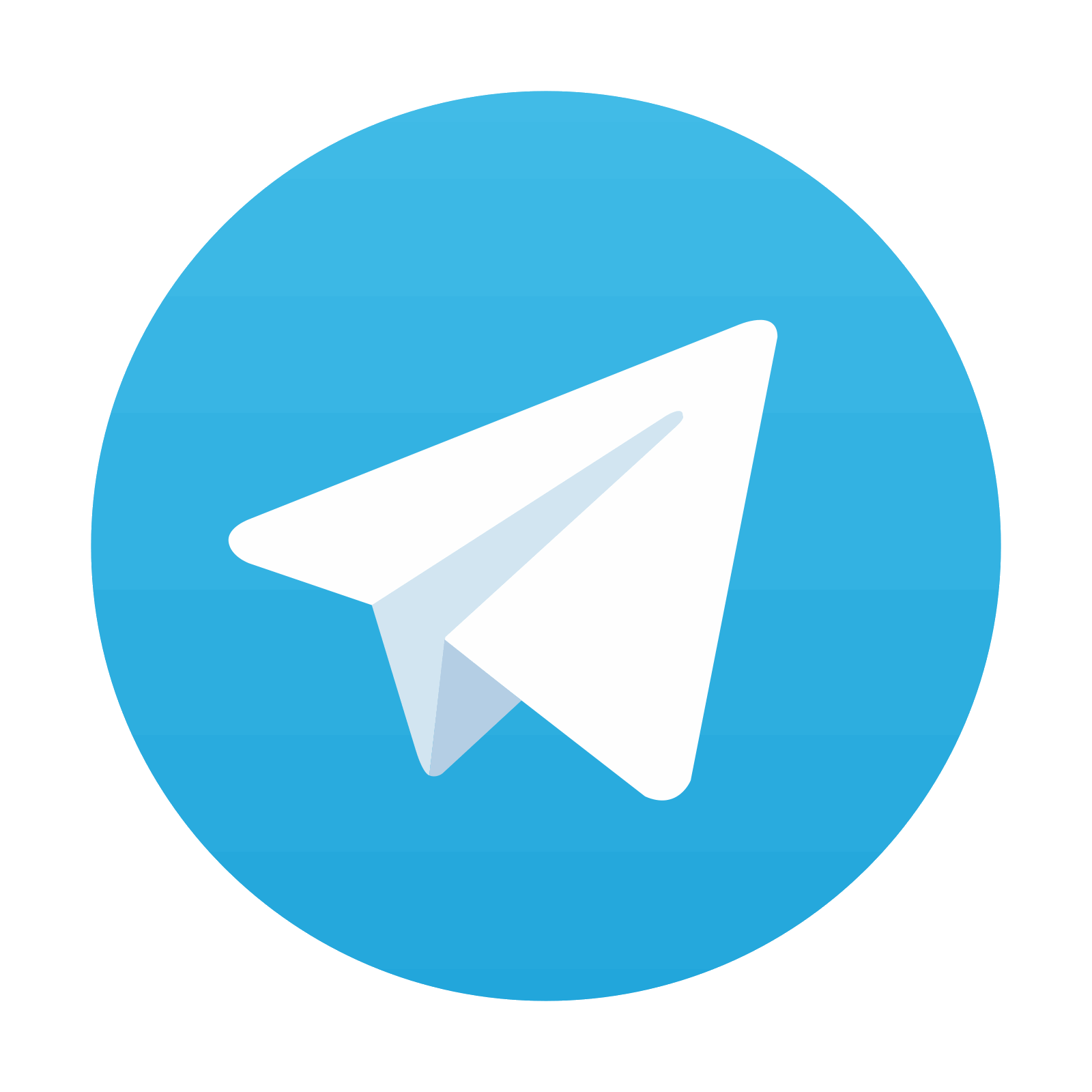
Stay updated, free articles. Join our Telegram channel
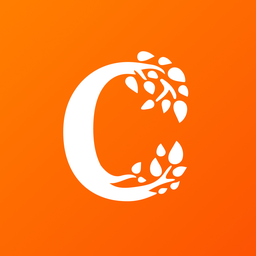
Full access? Get Clinical Tree
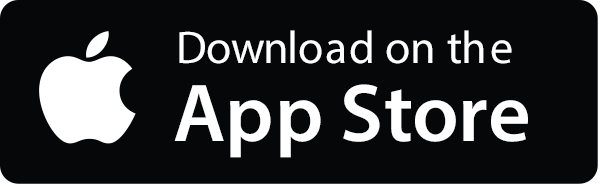
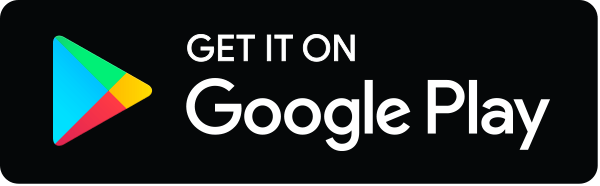