Fig. 27.1
The basic structure of laser includes a gain medium, a resonant optical cavity, and an external energy source which excites the gain medium. The resonant cavity is formed by two reflective mirrors. Mirror 1 is 100% reflective, while Mirror 2 is partly transparent and allows laser light to emit from the cavity
The basic cavity arrangement mentioned above allows continuous emission of laser energy. For laser lithotripsy, instead of using continuous wave laser, laser light is generally released in a pulsed fashion by various mechanisms, e.g., Q-switching, mode-locking, pulsed pumping, etc. The pulse duration plays an important role in deciding the mechanism of lithotripsy. The pulse duration for lithotripsy varies from femtoseconds to milliseconds, and is generally categorized as ultrashort-pulsed (less than 500 ns), short-pulsed (1–10 μs), and long-pulsed (greater than 250 μs) [2].
Physics of Laser Lithotripsy
Depending on the mechanism of laser–target interaction, there are three possible mechanisms: photochemical, photothermal, and photomechanical interactions. Photochemical interactions are characterized by the chemical reactions that are activated, facilitated, or catalyzed by the absorption of light by the target. Photochemical laser mechanism is not currently utilized for lithotripsy. Depending on the duration of laser pulse, photomechanical and/or photothermal interactions occur during laser lithotripsy. In short-pulsed laser lithotripsy (pulse duration ranging from several nanoseconds to several microseconds), the fragmentation of stones is mainly due to photomechanical laser–target interaction, while photothermal interaction is typically seen in long-pulsed laser lithotripsy (pulse duration is several hundred microseconds).
For a photomechanical interaction, the energy of the laser light generates plasma on the surface of the target or in the surrounding media immediately after the onset of laser. The plasma expands to a hemispherical bubble and then collapses or implodes within a few hundred microseconds. During this process, the energy of light is finally converted to mechanical energy in the form of shock waves traveling in all direction. Therefore, a photomechanical interaction is sometimes referred to as a photoacoustic interaction. In the specific application of laser lithotripsy, the pressure of laser-induced shock wave is typically higher than 100 bars. During the photomechanical-type laser lithotripsy, the kidney stones are finally fragmented by mechanical force. As expected, the ablation efficiency is dependent on the mechanical strength of the calculi. It is less efficient in fragmenting relatively hard or “durile” urinary calculi, such as calcium oxalate monohydrate and cystine stones [3]. Q-switched Nd:YAG laser (wavelength: 1,064 nm; pulse duration: tens of nanoseconds), Q-switched alexandrite laser (wavelength: 755 nm; pulse duration: hundreds of nanoseconds), and the flashlamp pumped pulsed dye laser (FPDL, wavelength: around 540 nm; pulse duration: several microseconds) all utilize photomechanical mechanism to fragment kidney stones [4–6]. These lasers produce relatively large fragments (diameter ranging from several hundred microns to several millimeters, fragments larger than 4 mm in diameter were found) during lithotripsy. Plasma is a specific state of matter created by generation of a shared electron cloud and is typically seen with extremely high peak powers created by ultrashort lasers. Not all photomechanical lithotripsy lasers require a plasma to generate photomechanical lithotripsy. In lasers with a pulse duration typically in the range of 1–10 μs, cavitation occurs without plasma formation, and photomechanical lithotripsy occurs.
For long-pulsed laser lithotripsy (pulse duration ranging from 250 to 700 μs), the fragmentation process is dominated by a photothermal mechanism. During a photothermal laser lithotripsy process, a small portion of laser energy is first absorbed by the surrounding media (i.e., water) between fiber tip and stone surface. Water is locally evaporated and forms a local vapor channel. This phenomenon is called the Moses Effect (as in Moses parting the Red Sea). The resulting vapor bubble expands and then collapses, producing weak pressure transients, typically nine bars or less. The magnitude of the pressure transient is significantly weaker than that produced in a photomechanical laser–target interaction and is not capable of fragmenting the stone. The ablation is achieved by the remaining laser energy which is transmitted through the vapor bubble and absorbed directly by the stone. During the 100-μs pulse duration, the laser-induced heat is not able to diffuse away, and is well confined within the irradiation area, causing disruption of the stone lattice and debris ejection. Examples of photothermal interaction include Holmium:YAG (Ho:YAG) and Erbium:YAG (Er:YAG) lithotripsy. Unlike short-pulsed lasers, these long-pulsed lasers are effective for all stone compositions (including durile compositions) and produce relatively small fragments.
Besides photochemical, photomechanical, and photothermal interactions, an emerging approach to efficient stone fragmentation is to harness the multiphoton absorption effects with femtosecond lasers. This technique is currently only available in research laboratories [7]. Although femtosecond laser fires ultrashort laser pulses, femtosecond ablation is characterized by tiny fine debris similar to long-pulsed laser ablation. The ablation process is governed by a plasma-mediated mechanism and is fundamentally distinct from a photomechanical mechanism. During the femtosecond laser lithotripsy process, the calculus is ionized and plasma is generated (i.e., mixture of ions and electrons). After that, incident laser energy is absorbed by free electrons in plasma, exciting electrons and creating additional free electrons [8]. The increased number of high-speed free electrons brings up the local temperature of the stone and disintegrates the stone. Femtosecond laser lithotripsy produces small-sized debris that is mostly less than 1 μm in diameter, significantly smaller than those produced in a photomechanical lithotripsy.
Current Gold Standard: Holmium:YAG Laser Lithotripsy
An ideal laser lithotripsy device should be capable of fragmenting all stone compositions and has a wide safety margin for surrounding tissues. The stone fragments produced from lithotripsy should be reasonably small in size so that they can be passed spontaneously. The fragmentation process should be efficient in order to shorten the duration of surgery. Generally speaking, photothermal lasers provide less efficient fragmentation (slower fragmentation over a longer period of time) than photomechanical lasers [9]. However, photothermal lasers produce smaller fragments and fragment all stone compositions.
One concern during all laser lithotripsy is stone retropulsion. During lithotripsy, stone fragments eject from the surface of a stone. Due to conservation of momentum, the stone moves in the opposite direction of fragment ejection, resulting in stone retropulsion. Strong retropulsion significantly slows down lithotripsy process because the surgeon has to move the endoscope and fiber to the new stone position after laser discharge and stone displacement, potentially making lithotripsy more difficult. Photomechanical lasers generally produce stronger retropulsion than photothermal lasers.
The long-pulse Ho:YAG laser (pulse durations of 250–700 μs, wavelength of 2.1 μm) has been used for intracorporeal laser lithotripsy of urinary calculi since the 1990s, and is considered to be the gold standard modality for laser lithotripsy [10–17]. As mentioned above, the laser–target interaction mechanism for Ho:YAG laser lithotripsy is photothermal [18]. The laser produces small-sized fragments [10], fragments all stone compositions, and causes less stone retropulsion compared with short-pulsed lasers [19, 20]. It is clinically safe because Ho:YAG light is highly absorbed by water, reducing collateral damage.
As a trade-off for the production of smaller stone fragments, Ho:YAG lithotripsy is less efficient and requires longer procedure time than photomechanical lasers [15]. The efficiency of Ho:YAG lithotripsy can be improved by increasing laser energy or pulse frequency, but increased pulse energy comes at the cost of larger fragments, increased retropulsion, and fiber tip degradation with energy settings >1.0 J [21]. Thus, the optimal Ho:YAG power setting is largely a balance among fragmentation efficiency, maintaining small stone fragments, and reducing retropulsion.
Previous study shows that retropulsion is greater for larger diameter fibers at a given energy or for larger energies for a given fiber diameter [22, 23]. The relationship of retropulsion on fiber diameter is illustrated in Fig. 27.2. A large-diameter fiber produces a wide and shallow crater, while a thin fiber produces a narrow and deep one. Since stone fragments eject at a normal incident to stone surface, the ejection of fragments from a wide and shallow crater is more uniform in the direction off the stone surface, producing greater momentum to the stone in the opposite direction. Another solution to minimize retropulsion is to use longer pulse duration. A longer pulse duration Ho:YAG laser achieves less retropulsion per pulse, and less retropulsion per unit of stone ablation compared to the “normal” long-pulse Ho:YAG laser [24, 25]. One possible reason is the occurrence of recoil pressure during debris ejection, which is proportional to the radiant power (energy per pulse duration) [26]. As a result, shorter pulse durations with high energy density produce higher retropulsion. To sum up, stone retropulsion during Ho:YAG laser lithotripsy can be reduced by decreasing fiber diameter and increasing the pulse duration.
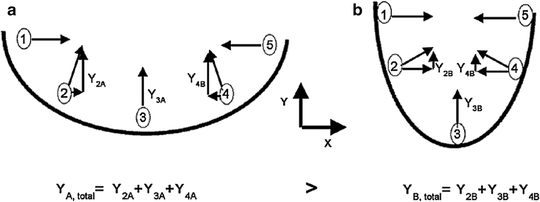
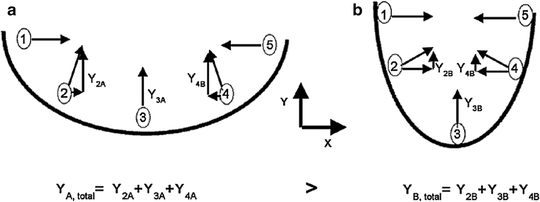
Fig. 27.2
Retropulsion is dependent on the size of optical fiber. (a) A wide optical fiber produces a wide and shallow crater with more retropulsion; (b) a narrow optical fiber produces a narrow and deep crater with less retropulsion. Reprinted from Lee et al. [22] with permission from Elsevier Limited
Clinically, metal-based stabilization or anti-retropulsion devices may be used to reduce retropulsion. The anti-retropulsion device is delivered proximal to the stone before laser energy is applied and prevents retropulsion. Such devices are effective but add operative expense. Metal devices can be damaged potentially by Ho:YAG laser energy. Recently, a reverse thermosensitive material has been proposed as an alternative approach of fixing stones during lithotripsy [27, 28]. The thermosensitive polymer is solid at physiological temperature (37 °C) but becomes liquid when the temperature is lower than 20 °C. Before laser lithotripsy, the polymer is delivered above the stone under low temperature, and thus solidifies as a gel plug at body temperature. During lithotripsy, the solid polymer prevents stone retropulsion. After the laser operation, it can be removed via conventional irrigation. Comparing metal-based anti-retropulsion devices to the reverse thermosensitive polymer, all anti-retropulsion devices here are effective. Some potential advantages of the reverse thermosensitive polymer: it requires no wire to control its position during laser operation, so it does not interfere with the lithotripter and allows a free operative field. In addition, it retains its structure and anti-retropulsion function even if targeted by laser energy.
The ideal power parameters of Ho:YAG lithotripsy are incompletely understood. The efficiency of Ho:YAG lithotripsy can be improved by increasing pulse energy or pulse frequency, both of which increase power. Increased pulse energy produces more lithotripsy per pulse but also produces increased retropulsion and larger stone fragments. Stone retropulsion reduces lithotripsy efficiency because it decouples the fiber tip from the stone surface and increases energy that is absorbed by water between laser tip and stone surface, rather than the stone itself. Prior studies of Ho:YAG laser lithotripsy efficiency favor pulse energy settings less than 1.0 J at high repetition rate [21, 29]. In a recent study, 2.0 J pulse energy setting produces more than four times as much fragmentation as the 0.2 J setting, both at 10 Hz, with only a marginal increase in fragments larger than 1 mm [30]. Total fragmentation (defined as the initial stone mass minus the dominant residual fragment mass) increases as Ho:YAG pulse energy increases. Low pulse energy at a fast repetition rate produces fine, powderized fragmentation debris but overall a slower fragmentation rate compared to using high pulse energy settings at the risk of larger fragmentation chunks. Urologists should select lithotripsy power settings according to desired consequent outcomes. For example, a urologist may want to choose high pulse energy settings to fragment large bladder stones which require lengthy operative time devoted to laser lithotripsy and present less retropulsion due to their large mass, while for smaller stones where retropulsion is the main concern, pulse energy should be turned down to perform a careful ablation with minimal retropulsion and production of tiny debris in the ureter. Anti-retropulsion devices are found to improve the efficiency of lithotripsy, particularly at high pulse energy [30]. With ureteral stone stabilization, high pulse energy settings fragment a stone much more quickly than achieved at low pulse energy settings as retropulsion is negated.
Optical Fibers
Optical fibers are utilized in laser lithotripsy to transmit laser radiation to the calculi with high efficiency. The basic structure of optical fiber consists of a transparent inner core, two to three layers of cladding with lower refractive indices, a buffer layer, and a surrounding jacket. Due to total internal reflection, light will transmit along the fiber core with very low attenuation.
Silica (amorphous silicon dioxide, SiO2) is the most widely used material for the core. They are clinically used in laser lithotripsy because they are relatively cost-efficient, small, and flexible enough for endoscopic instruments, and they transmit laser light with minimal attenuation. Ho:YAG laser light at 2.1 μm wavelength is highly absorbed by hydroxyl groups; thus it is delivered by low OH silica fiber to reduce light loss and avoid fiber damage [31]. Diameter of the fiber core used for lithotripsy is variable from 150 to 940 μm, normally much smaller than diameter of the collimated laser beam from the laser source. For Ho:YAG wavelengths, fluorine-doped silica which has excellent laser light confinement and small bending radius is the main choice of cladding materials [32].
The laser light irradiated from the laser device is usually collimated, and the diameter of laser beam is often much larger than that of the optical fiber. In order to deliver laser light to the optical fiber, laser light is focused by a convex lens and coupled into the proximal optical fiber tip. To maximize the amount of laser light that is coupled into the fiber, the light has to be focused to the fiber tip with an acceptable divergence angle. In this process, two beam shape parameters are critical to obtain high-efficiency laser–fiber coupling: diameter and numerical aperture (NA) [32, 33]. The diameter of the focused laser beam at fiber surface, or beam waist, should be smaller than the diameter of fiber core to avoid light leakage. However, if the beam diameter is too small, the high energy of the laser light could possibly damage the optical fiber. Thus, a sufficiently large, but smaller than fiber core, beam size is required [34]. The other important parameter, NA, determines the maximal divergence angle that allows a beam to enter the optical fiber [32]. The transmission of light along optical fiber relies on total internal reflection at the core–cladding interface. If the incident angle is larger than the total reflection angle, the light can penetrate the fiber cladding and possibly damage the fiber. The NA of Ho:YAG fibers is 0.2–0.22 [34]. An NA of 0.21 corresponds to a maximum acceptance angle of 12°. The focusing lens should match the NA of optical fiber.
Commercially, an industry standard subminiature version A (SMA) connecter is connected to the proximal end of optical fiber so that fibers with different diameters can easily connect to the same laser source. An SMA connector is designed with an air-filled cavity surrounding the proximal end of the fiber to protect from leakage-related damage [32]. The cavity is made of a polymer material and the shell is usually composed of steel. The SMA connecter protects the optical fiber from mechanical damage and facilitates the alignment of laser–fiber coupling. However, even with the help of SMA connecter, there may still be some axial misalignment of laser and proximal fiber end, especially for small-diameter fibers with core diameter less than 300 μm, which can result in higher order rays, energy attenuation, or fiber damage. For large core fibers, a small misalignment of laser beam from the center of fiber core is sometimes acceptable as long as the beam is focused within the fiber core, but for small-diameter fibers, the partial overlap between laser beam and fiber cladding results in light leakage and fiber damage. Under high power settings, the steel shell of SMA connecter can be vaporized by the energy of stray laser which is not fully coupled into the optical fiber. Over time, the steel vapor will coat the surface of optical fiber and laser source and disrupt their optics structure, leading the failure of laser.
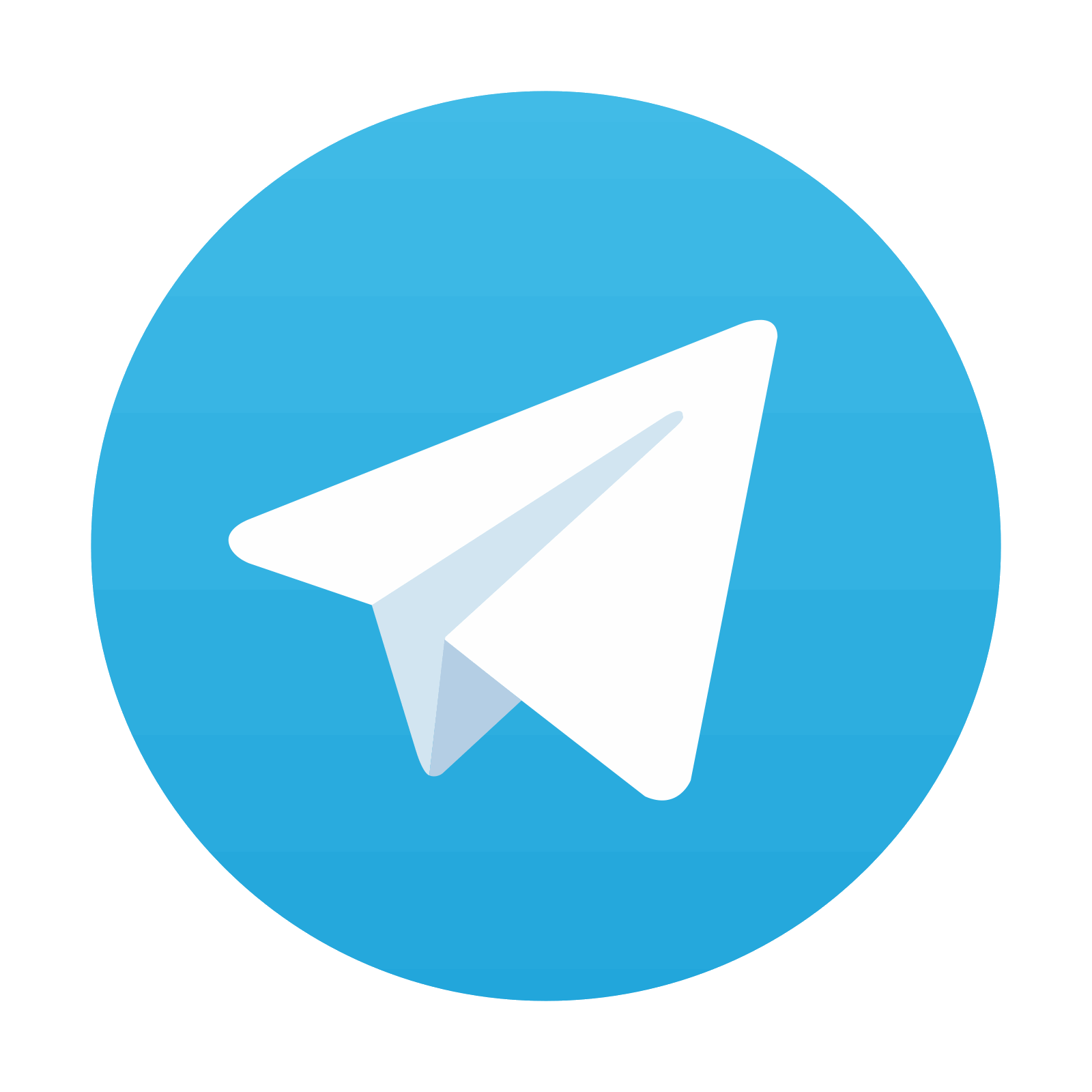
Stay updated, free articles. Join our Telegram channel
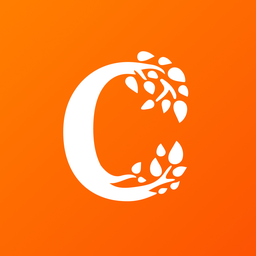
Full access? Get Clinical Tree
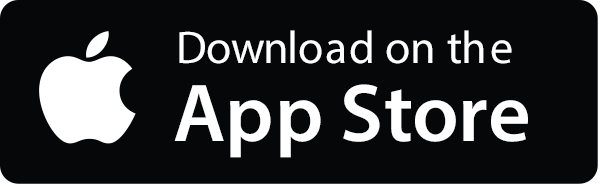
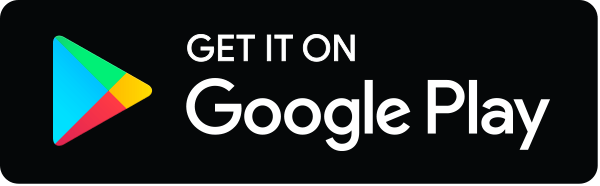