Abstract
The water-soluble vitamins represent a group of structurally and functionally unrelated compounds that share the common feature of being essential for normal cellular functions, growth, and development. With the exception of some endogenous synthesis of vitamin B 3 (niacin), human cells cannot synthesize these micronutrients, and thus, must obtain them from exogenous sources via intestinal absorption. The intestine, therefore, plays a critical role in maintaining and regulating normal body homeostasis of these essential micronutrients. Interference with the normal intestinal absorption process of these vitamins leads to suboptimal/deficiency states and development of metabolic derangements and clinical abnormalities. Impairment in intestinal absorption of water-soluble vitamins occurs in a variety of conditions including genetic defects (mutations) in the digestive or absorptive systems involved, intestinal diseases, chronic alcohol use, infection with certain enteropathogens, and drug-vitamin interaction.
Keywords
Vitamin B 1 (thiamin), Vitamin B 2 (riboflavin), Vitamin B 3 (niacin), Vitamin B 5 (pantothenic acid), Vitamin B 6 (pyridoxine), Vitamin B 7 (biotin), Vitamin B 9 (folate), Vitamin B 12 (cobalamin), Vitamin C (ascorbic acid, dehydro-ascorbic acid), Transport mechanisms, Transport regulation
Abbreviations
AMV
apical membrane vesicles
AA
ascorbic acid
BLM
basolateral membrane
BLMV
basolateral membrane vesicles
BBM
brush-border membrane
BBMV
brush-border membrane vesicles
CaM
Ca/calmodulin
ChIP
chromatin immunoprecipitation
Cbl
cobalamin
cKO
conditional Knockout
DHAA
dehydro- l -ascorbic acid
DIDS
4,4′-diisothiocyano-2,2′-disulfonic acid stilbene
EMSA
electromobility shift assay
EGFP
enhanced green fluorescent protein
EPEC
enteropathogenic Escherichia coli
ETEC
enterotoxigenic Escherichia coli
FAD
flavin adenine dinucleotide
FMN
flavin mononucleotide
Glut2
glucose transporter-2
Glut8
glucose transporter-8
GST
glutathione S -transferase
HFMS
hereditary folate malabsorption syndrome
hnRNA
heterogeneous nuclear ribonucleic acid
IBD
inflammatory bowel disease
IFN-γ
interferon-γ
miRNA
microribonucleic acid
NAD
nicotinamide adenine dinucleotide
NADP
nicotinamide adenine dinucleotide phosphate
PKA
protein kinase-A
PKC
protein kinase-C
PTK
protein tyrosine kinase
PCFT
proton-coupled folate transporter
PDZD11
PDZ domain containing 11
RFC
reduced folate carrier
RF
riboflavin
RFVT-2
riboflavin vitamin transporter-2
RFVT-3
riboflavin vitamin transporter-3
RFVT-1
riboflavin vitamin transporter-1
siRNA
small interfering ribonucleic acid
SMVT
sodium-dependent multivitamin transporter
SVCT-1
sodium-dependent vitamin C transporter-1
SVCT-2
sodium-dependent vitamin C transporter-2
TNF-α
tumor necrosis factor-α
TPP
thiamin pyrophosphate
TPPT
thiamin pyrophosphate transporter
TRMA
thiamin-responsive megaloblastic anemia
THTR-1
thiamin transporter-1
THTR-2
thiamin transporter-2
TJ
tight junction
TCN
transcobalamin
54.1
Introduction
The water-soluble vitamins represent a group of structurally and functionally unrelated compounds ( Fig. 54.1 ) that share the common feature of being essential for normal cellular functions, growth and development. With the exception of some endogenous synthesis of vitamin B 3 (niacin), human cells cannot synthesize these micronutrients, and thus, must obtain them from exogenous sources via intestinal absorption. The intestine, therefore, plays a critical role in maintaining and regulating normal body homeostasis of these essential micronutrients. Interference with the normal intestinal absorption process of these vitamins leads to sub-optimal/deficiency states and development of metabolic derangments and clinical abnormalities. Impairment in intestinal absorption of water-solbule vitamins occurs in a variety of conditions including gentic defects (mutations) in the digestive or absorptive systems involved, intestinal diseases, chronic alcohol use, infection with certain enteropathogens, and drug-vitamin interaction.

Significant progress has been made in recent years in our understanding of the cellular and molecular mechanisms involved in intestinal absorption of water-soluble vitamins and how these events are regulated. We now know that absorption of physiological concentrations of all these micronutrients is mediated via specific carrier-mediated mechanisms. In many cases the molecular identity of the systems involved ( Fig. 54.2 ), their cell biology, and their regulation have been delineated. We also know now that the large intestine possess specialized carrier-mediated systems for the absorption of the microbiota-generated water-soluble vitamins ( Fig. 54.3 ) and that this source of vitamins controbute toward overall host nutriton, and especially toward the cellular nutrition of the local colonocytes. Our aim in this chapter is to provide a comprehensive description of the current understanding of the physiology and cellular/molecular biology of intestinal absorption of water-soluble vitamins, and how external/environmental conditons and internal factors affect and interfer with these events.


54.2
Vitamin B1 (Thiamin)
54.2.1
Metabolic Role and Effect of Deficiency
Thiamin ( Fig. 54.1 ) was the first member of the family of water-soluble vitamins to be described, with reference to the effects of its deficiency (beriberi) being suggested some 4000 years ago in old Chinese medical literatures. Thiamin (mainly in its pyrophosphate forms) acts as an enzymatic cofactor for transketolase, pyruvate dehydrogenase, α-ketoglutarate dehydrogenase, and branched-chain ketoacid dehydrogenase, enzymes involved in many critical metabolic reactions that relate to energy metabolism. Because thiamin bridges the glycolytic and the pentose phosphate metabolic pathways (which generate chemical-reducing power in cells), it also plays an important role in reducing cellular oxidative stress. Thus, low intracellular levels of thiamin will lead to impairment in energy metabolism (acute energy failure) and to a propensity for oxidative stress. While most of thiamin’s biological activity is attributed to its pyrophosphate form (i.e., thiamin pyrophosphate, TPP, which is also the most abundant form of this vitamin in cells and accumulates mainly in mitochondria), recent studies have shown that other forms of the vitamin, namely, thiamin triphosphate, also possess biological activity in nerve cells where it regulates the function of membrane chloride channels and acts as a phosphate group donor to other membrane proteins (Ref. and references therein). Thiamin deficiency (and suboptimal levels) represents a significant nutritional problem worldwide and leads to several clinical abnormalities including neurological (neuropathy and/or Wernicke-Korsakoff syndrome) and cardiovascular (peripheral vasodilatation, biventricular myocardial failure, edema, and potentially acute fulminant cardiovascular collapse) disorders. On the other hand, optimization of thiamin levels appears to have the potential of preventing diabetic retinopathy and tissue damage caused by the hyperglycemia of diabetes and alleviating fatigue in patients with inflammatory bowel diseases (IBDs). Systemic thiamin deficiency and suboptimal levels occur in a high percentage of chronic alcoholics, diabetics, patients with celiac sprue and renal diseases, post bariatric surgery, patients with sepsis, and in subjects on long-term furosemide diuretic therapy. In addition, thiamin deficiency and suboptimal levels occur in the elderly population despite an average daily intake of the vitamin that exceeds their recommended requirement. Also recognized is the occurrence of thiamin deficiency disorders that occur despite normal plasma level of this vitamin. Examples of the latter are thiamin-responsive megaloblastic anemia (TRMA) and thiamin-responsive/Wernicke’s-like encephalopathy. TRMA is an autosomal recessive disorder characterized by megaloblastic anemia, sensorineural deafness, and non-type 1 diabetes mellitus, which is caused by mutations in the human thiamin transporter-1 (hTHTR-1; product of the SLC19A2 gene). Such mutations in hTHTR-1 gene led to an impairment in thiamin uptake (by the affected tissues) and the development of localized thiamin deficiency. Thiamin-responsive/Wernicke’s-like encephalopathy is characterized by seizures, ophthalmoplegia, nystagmus, and ataxia and is believed to be due to mutations in the human thiamin-transporter-2 (hTHTR-2; product of the SLC19A3 gene). In both of the latter disorders, oral administration of pharmacological doses of thiamin brings about significant improvements in many of the clinical symptoms of the affected individuals.
54.2.2
Physiology of the Intestinal Thiamin Absorption Process
The human intestine encounters thiamin from two sources: a dietary source and from microbiota. Dietary thiamin exists mainly in the phosphorylated form, which must be hydrolyzed to free thiamin prior to absorption (the small intestine does not have a transport system for intact TPP, that is, it is unlike the large intestine that has such a system; see below), a process that is achieved by the action of the abundant intestinal phosphatases. Absorption of the liberated free thiamin (which exists in the monocationic form at gut luminal pH) then takes place mainly in the proximal part of the small intestine. The mechanism of thiamin absorption in the small intestine has been investigated using a variety of intestinal preparations from different species including human. Collectively, these studies have shown the involvement of a specific carrier-mediated mechanism that is inhibited by thiamin structural analogues, but not by unrelated organic cations. Studies using purified brush-border membrane vesicles (BBMV) isolated from human and animal small intestine have shown the involvement of a pH (but not Na + )-dependent, electroneutral, and amiloride-sensitive carrier-mediated mechanism for thiamin uptake. The mechanism by which thiamin exits out of enterocytes, that is, transport across the basolateral membrane (BLM), has also been studied using purified human and animal intestinal basolateral membrane vesicles (BLMV) and has been found to involve a specific, pH-dependent, electroneutral, carrier-mediated process. With regard to potential metabolism during absorption, studies have shown that some of the absorbed free thiamin is rephosphorylated (mainly to TPP) in intestinal epithelial cells; however, only free thiamin exits the intestinal absorptive cells into the serosal side. There is recent emerging evidence that indicates that the intracellularly generated TPP accumulates in mitochondria via a carrier-mediated process and this important process has major relevance to intestinal energy metabolism in health and disease.
As to the microflora-generated thiamin, this source provides considerable amounts of free and phosphorylated (TPP) forms of the vitamin. While gut microbiota of all human generate thiamin, recent studies that classified the human gut microbiota into three functionally different enterotypes (enterotypes 1, 2, and 3) have reported that enterotype 2 (which is enriched in Prevotella and Desulfovibrio ) is especially overrepresented in enzymes that are involved in TPP biosynthesis, implying a larger level of thiamin generation in the group that harbors this enterotype. As to absorbability of the microbiota-generated forms of thiamin in the large intestine, recent investigations have shown that the human colonocytes do indeed possess an efficient and specific carrier-mediated mechanism for uptake of free thiamin and a separate and specific carrier-mediated mechanism for uptake of intact TPP. The latter system was shown to have high affinity (apparent K m of 0.157 μM) and specificity for TPP (it does not transport free thiamin or thiamin monophosphate), is pH- and Na + -independent, and energy-dependent. It is also interesting to note that the gene that encodes the colonic TPP transporter (TPPT) (i.e., SLC44A4; see below) has recently been identified as an ulcerative colitis susceptibility gene. Based on the mentioned evidence, it is clear that the microbiota-generated thiamin is absorbable and contributes to overall thiamin nutrition, especially when it come to cellular nutrition and health of the local colonocytes.
54.2.3
Molecular Biology of the Intestinal Thiamin Uptake Systems
Mammalian cells appear to utilize two members of the solute carrier SLC19A gene family (i.e., THTR-1 and THTR-2) to transport free thiamin and one to transport TPP. The SLC19A2 encodes a protein of 497 amino acids (THTR-1), while the SLC19A3 encodes a protein of 496 amino acids (THTR-2). Both of the human THTR-1 and -2 are predicted to have 12 putative transmembrane domains (TMDs) with their N- and C-terminal tails extending into cell interior. The hTHTR-1 and hTHTR-2 share 48% identity and 64% similarity with one another. They also share 40% and 39% identity at the amino acid level, respectively, with the human reduced folate carrier (hRFC). However, neither hTHTR-1 nor hTHTR-2 transport folate and the hRFCs do not transport free thiamin.
The hTHTR-1 appears to function in the micromolar range, while the hTHTR-2 appears to function in the nanomolar range. Both the hTHTR-1 and hTHTR-2 are expressed in the human small and large intestine, with expression of the former being markedly higher than that of the latter. The membrane domain of the polarized human enterocyte at which hTHTR-1 and hTHTR-2 proteins are expressed have also been investigated using immunological and confocal imaging approaches. Expression of the hTHTR-1 protein was shown to be at both the apical and BLM domains of the polarized enterocytes, with a slightly higher expression at the latter compared to the apical membrane domain. On the other hand, expression of the hTHTR-2 protein was shown to be restricted to only the apical membrane domain of the polarized absorptive epithelia.
As to the molecular identity of the colonic TPPT, this has been recently delineated in studies by Nabokina et al., which showed that the uptake system is the product of the SLC44A4 gene. Expression of the human TPPT along the intestinal tract was found to be restricted to the colon ( Fig. 54.4 ). Also, cell surface biotinylation assay and live-cell confocal imaging studies have shown that the human TPPT is exclusively expressed at the apical membrane domain of polarized epithelial cells ( Fig. 54.5 ). The tissue-specific expression of the TPPT in the colon appears to be determined by epigenetics as well as microribonucleic acid (miRNA)-mediated mechanisms. The latter was suggested by findings that in the colon, histone H3 in the 5′-regulatory region of Slc44a4 is trimethylated at lysine 4 and acetylated at lysine 9, whereas the trimethylation at lysine 27 was negligible. In the jejunum, on the other hand, histone H3 was found to be hypertrimethylated at lysine 27 (repressor mark). Involvement of miRNA(s) in the tissue-specific expression of TPPT was suggested by findings showing the 3′-UTR of SLC44A4 to be the target of specific miRNAs/RNA-binding proteins in noncolonic, but not in colonic, epithelial cells.


54.2.3.1
Role of THTR-1 and -2 in Intestinal Thiamin Uptake
Since the hTHTR-1 and -2 are both expressed in intestinal epithelial cells, it was important to understand their relative contribution toward total carrier-mediated intestinal thiamin uptake. This was done with the use of two approaches: (i) selective knockdown of hTHTR-1 and -2 with gene-specific small-interfering RNAs (siRNAs) and (ii) use of THTR-1 and -2-knockout mouse models. Results of the first approach showed that both the hTHTR-1 and -2 are involved in intestinal thiamin uptake and that together they account for total carrier-mediated thiamin uptake across the apical membrane of human intestinal epithelial cells. Results of the second approach showed a significant and specific reduction in thiamin uptake by the intestine in the THTR-2-knockout mice compared to uptake by the intestine of their sex-matched wild-type littermate. This was also associated with a significant reduction in blood thiamin levels in the knockout animals when compared to the control group. However, thiamin uptake by the intestine in THTR-1-knockout mice was not significantly different from that of their sex-matched wild-type littermates. Furthermore, the level of expression of THTR-1 was not altered in the intestine of THTR-2-knockout mice, while the level of expression of THTR-2 was markedly upregulated in the intestine (and kidney) of THTR-1-knockout mice. These findings show that THTR-2 is required for normal thiamin uptake in the intestine. The data also provided an explanation as to why patients with TRMA (who have a mutated and dysfunctional hTHTR-1) have normal plasma levels of thiamin. This is most likely due to an induction in the level of expression of hTHTR-2 in the intestine (and kidney), which leads to a higher intestinal uptake (and renal reuptake) of thiamin.
54.2.4
Cell Biology of the Intestinal Thiamin Absorption Process
Insight into the mechanisms involved in intracellular trafficking and membrane targeting of the human thiamin transporters in epithelial cells have also been emerging from studies utilizing the live-cell confocal imaging approach. Using a series of truncated hTHTR-1 constructs fused with the enhanced green fluorescent protein (EGFP) (i.e., hTHTR1-EGFP), expression of the full-length fusion protein was shown to be at both the apical and the BLM domains of intestinal epithelial cells. Analysis of the localization pattern of truncated mutants of the hTHTR-1 has shown that while the C-terminus region of the polypeptide does not appear to have a role in membrane targeting, whereas an essential role for the N-terminal and the backbone was found. Also, truncation of the hTHTR-1 polypeptide within a region where several TMRA truncations are clustered results in intracellular retention of the mutant protein. As to intracellular trafficking, the hTHTR-1 protein was found to reside inside numerous trafficking vesicles whose movement is temperature dependent and requires an intact microtubule network for their mobility (Ref. ; real-time movies can be viewed at website: http://www.jcb.org/cgi/content/full/278/6/3976/DC1 ).
With regard to the hTHTR-2 protein, this carrier is expressed exclusively at the apical membrane domain of the polarized intestinal epithelial cells. Live-cell imaging investigations utilizing a series of hTHTR-2 truncations have shown that while the cytoplasmic C-terminal is not essential for membrane targeting of the protein, an essential role for the transmembrane backbone is evident. In addition, trafficking vesicles were found to be involved in the intracellular movement of hTHTR-2 to the cell surface, and that mobility of these vesicles depends on an intact microtubule network that was disrupted by overexpression of the dynactin subunit dynamitin (p50) (dynactin is involved in trafficking of vesicles via conventional cytoplasmic dynein, a minus end-directed motor protein).
Other studies have identified accessory proteins that influence the functionality and cell biology of the hTHTR-1 and -2 in intestinal epithelial cells ( Fig. 54.6 ). Using a bacterial two-hybrid system to screen a human intestinal cDNA library with the complete coding sequence of hTHTR-1 as bait, studies have identified a member of the tetraspanin family of proteins, Tspan-1, as an interacting partner with hTHTR-1. Coimmunoprecipitation assay, glutathione S -transferase (GST)-pulldown assay, and live-cell confocal imaging have confirmed the existence of such an interaction between hTspan-1 and hTHTR-1 in human intestinal epithelial Caco-2 cells. This interaction appears to have biological and functional consequences as it decreased the rate of degradation of the hTHTR-1 protein, and thus, influenced its functionality. More recent investigations have utilized a yeast split-ubiquitin two-hybrid approach to identify the human transmembrane 4 superfamily 4 (hTM4SF4) as an interacting partner of hTHTR-2 in human intestinal epithelial cells ( Fig. 54.6 ). This interaction was again confirmed by GST-pull-down assay in vitro , by live-cell confocal imaging in intestinal epithelial cells, and shown to have functional consequences on the hTHTR-2 activity.

54.2.5
Structure-Activity Aspects of the Thiamin Transporters
Knowledge about the structure-function activity of the hTHTR-1 and hTHTR-2 proteins has been emerging in recent years from clinical and experimental findings. With regard to the hTHTR-1, over 18 different clinical mutations have been identified in patients with TRMA. These mutations are either mis-sense or nonsense in nature with the latter type leading to an early truncation of the carrier protein. The effect of a number of these mutations (e.g., P51L and T158R, W358X, and Delta383fs) on functionality of the hTHTR-1 has been investigated experimentally using mutants generated by site-directed mutagenesis followed by expression in intestinal and other appropriate cell types. The results revealed a spectrum of mutant phenotypes with all exhibiting impaired thiamin uptake function due to either a change in hTHTR-1 level of expression/stability, mis-targeting, and/or altered transport activity. Other studies have examined the role of the only conserved anionic amino acid residue (located at position 138) in the predicted TMDs of hTHTR-1 in the transport of the cationic thiamin, as well as the role of the two putative N-glycosylation sites (predicted to be at positions 63 and 314). The result showed a critical role for the residue at position 138 in the function of hTHTR-1. On the other hand, neither of the putative glycosylation sites was found to play a role in the function or membrane targeting of the hTHTR-1 protein.
As to the hTHTR-2 protein, again data from clinical and experimental studies have shed light into important aspects of its structure-activity relationship. Two clinical mutations (K44E and E320Q) in hTHTR-2 have been described in patients with thiamin-responsive Wernicke’s-like encephalopathy. Experimental testing of these two mutants showed impaired functionality of hTHTR-2 in both cases. The K44E mutant caused thiamin transport dysfunction by impairing hTHTR-2 trafficking to the cell membrane. Another two mutations in the hTHTR-2 protein (G23V and T422A) have been reported in patients with biotin-responsive basal ganglia disease (although it is unclear at present as to why mutations in a specific thiamin transporter precipitates this condition despite the fact that hTHTR-2 does not transport biotin; Ref. ). Both of these mutations in hTHTR-2 were found to cause functional impairment, with no defect in the expression of this transporter at cell membrane. It is interesting to mention here that both of the affected residues (i.e., at positions 23 and 422) are conserved in the hTHTR-2 sequences of all species cloned so far, as well as in other members of the SLC19A gene family, that is, the hTHTR-1 and the (hRFC; product of the SLC19A1 gene), thus, supporting an essential role of these residues in transporter functionality. Hydropathy analyses suggested that these residues (G23V in TM1, T422A in TM11) lie within the TMDs of the hTHTR-2 protein. Crystallographic data from other major facilitator superfamily transporters showed that both TM1 and TM11 contribute to the central hydrophilic cavity of the transporters, underscoring the likelihood that mutation of residues within these regions could impair transporter function (reviewed in Ref. ). Other studies have examined the role of the three negatively charged conserved glutamic acid residues within the TMDs of hTHTR-1 and hTHTR-2 (i.e., E120, E320, and E346) as well as the role of the two putative N-glycosylation sites in the hTHTR-2 polypeptide (i.e., N45, N166) in the function and cell biology of the hTHTR-2 protein. Results of these investigations showed that mutating any of the conserved glutamic acid residues lead to a significant impairment in transport of the positively charged thiamin. Furthermore, while mutating E120 and E320 led to a decrease in the level of expression of hTHTR-2 at the apical membrane domain of intestinal epithelial cells, mutating E346 did not affect this parameter. Finally, the two putative glycosylation sites of the hTHTR-2 polypeptide (i.e., N45Q, N166Q) do not appear to affect the functionality or level of expression of the hTHTR-2 in intestinal epithelial cells.
With regard to the colonic TPPT, recent studies have shown that the protein is glycosylated at positions N69, N155, N197, N393, and N416. However, only glycosylation at N69, N155, and N393 appears to be important for function, most likely through an effect on protein conformation and/or interaction with the substrate.
54.2.6
Regulation of the Intestinal Thiamin Uptake Process
The intestinal thiamin uptake process appears to be under the regulation of a variety of extracellular/environmental conditions and intracellular factors. Since a number of these conditions/factors exert their regulatory effect(s) at the transcription level, it is relevant to first describe the basal transcriptional regulation of the SLC19A2, SLC19A3 , and SLC44A4 genes.
54.2.6.1
Transcriptional Regulation of the SLC19A2, SLC19A3 , and SLC44A4 Genes
The 5′-regulatory region (promoter) of the SLC19A2 gene was cloned and characterized in human intestinal epithelial Caco-2 cells using the Firefly luciferase reporter gene assay. The minimal promoter region required for basal activity of the SLC19A2 promoter was shown to be encoded in a region between − 356 and − 36 (considering the A of the initiation ATG sequence as position 1) and include multiple putative cis-regulatory elements. A role for a number of these cis-elements (namely, GKLF, NF-1, and SP-1) has also been established in studies involving mutational analysis, oligonucleotide competition assays, and electromobility shift/supershift assays. Functionality and physiological relevance of the full-length and minimal SLC19A2 promoters were confirmed in vivo by expressing constructs of these promoters fused to the Firefly luciferase reporter gene in transgenic mice. The pattern of expression of these promoter constructs in different tissues was found to be similar to the pattern of expression of the hTHTR-1 mRNA in different human tissues. Other investigations have determined the transcription initiation sites of the SLC19A2 gene in intestinal epithelial Caco-2 cells using 5′-rapid amplification of cDNA ends, with three such sites being identified at positions − 183, − 192, and − 220. Finally, studies have reported that the human SLC19A2 promoter is a target for activation by the p53 tumor suppressor transcription factor in murine erythroleukemia cells, but it is not clear if a similar activation also occurs in intestinal epithelial cells.
With regard to the SLC19A3 gene, its promoter has been cloned and characterized in human intestinal epithelial cells in vitro, and its functionality confirmed in transgenic mice in vivo. The transcription initiation site of the SLC19A3 gene was found to be at position − 88, and the minimal promoter region required for basal activity was shown to be encoded in a sequence between − 77 and + 59 and contains a number of putative cis-regulatory elements. A critical role for the stimulating protein-1 (SP1)/guanosine cytidine box (GC-box)-binding site (located at position − 48/−45 bp) was established and both SP1 and SP3 appear to interact with this site. The latter was confirmed in Drosophila SL2 cells (which lack endogenous SP factors), where both SP1 and SP3 were found to transactivate the SLC19A3 minimal promoter in a dose-dependent manner. Functionality of the full-length SLC19A3 promoter was also confirmed in vivo in transgenic mice carrying the human SLC19A3 promoter-luciferase reporter gene, thus, establishing the physiological relevance of the in vitro studies.
As for the SLC44A4 gene, the 5′-regulatory region of this gene has been cloned and characterized in human colonic epithelial NCM460 cells. The minimal region required for basal activity of the SLC44A4 promoter was mapped to a sequence between nucleotides − 178 and + 88. Mutational analysis performed on putative cis-regulatory elements established a role for ETS/ELF3 [E26 transformation-specific sequence (ETS) proteins], cAMP-responsive element (CRE), and SP1/GC-box sequence motifs in basal activity of the SLC44A4 promoter. Binding of ELF3 and CRE-binding protein-1 (CREB-1) transcription factors to the SLC44A4 minimal promoter was also shown by means of electromobility shift assay (EMSA). Contribution of CREB to SLC44A4 promoter activity was confirmed using NCM460 cells overexpressing CREB. A higher expression of ELF3 and CREB-1 in colonic (NCM460) compared with noncolonic cells was also observed, which may suggest possible contribution of these factors to colon-specific pattern of SLC44A4 expression.
54.2.6.2
Adaptive Regulation of the Intestinal Thiamin Uptake Process
The intestinal thiamin uptake process appears to be adaptively regulated by extracellular thiamin levels. Thiamin deficiency in humans leads to an increase in thiamin uptake when compared to control subjects. This effect appears to be mediated via changes in the V max and the apparent K m of the intestinal thiamin uptake process. The finding of adaptive regulation in intestinal thiamin uptake process in thiamin deficiency has been confirmed in studies with mice, and in studies utilizing cultured human intestinal epithelial cells. In mouse studies, dietary thiamin deficiency was found to lead to significant induction in intestinal carrier-mediated thiamin uptake; this was associated with a significant increase in the level of expression of the mTHTR-2, but not the mTHTR-1. In addition, activity of the luciferase reporter gene in transgenic mice carrying the human SLC19A3 promoter-luciferase construct was found to be significantly higher in the intestine of thiamin-deficient mice compared to pair-fed transgenic controls. In contrast, no changes in the activity of the luciferase reporter gene in transgenic mice carrying the human SLC19A2 promoter-luciferase construct were observed during thiamin deficiency. This provides evidence that the adaptive upregulation in intestinal thiamin uptake process in thiamin deficiency is mediated via an induction in the level of expression of THTR-2 and that the induction is, at least in part, mediated via transcriptional mechanism(s). To further confirm the involvement of transcriptional mechanism(s) in the adaptive regulation of intestinal thiamin uptake and to delineate the molecular mechanism(s) involved, human intestinal epithelial cells (Caco-2 cells) were exposed to different levels of extracellular thiamin while transfected with different 5′-truncated promoter-luciferase constructs. These investigations showed that the thiamin level-responsive region in the SLC19A3 promoter is located between − 77 and − 29 (using transcriptional start site as + 1). In addition, a key role for SP1/GC-box in mediating the effect of extracellular thiamin level on SLC19A3 promoter was established. Furthermore, extracellular levels of thiamin were found to affect the level of expression of the SP1 protein and its binding to the thiamin level-responsive region in the SLC19A3 promoter.
It is worth mentioning here that different cells appear to utilize different mechanisms to upregulate their thiamin uptake. Thus, thiamin uptake in the kidney is upregulated in thiamin deficiency via induction in the level of expression of both THTR-1 and THTR-2, while in the brain and heart, the upregulation appears to be mediated via induction in the expression of THTR-1 mainly.
As to the colonic TPP uptake process, this event also appears to be adaptively regulated by extracellular substrate (TPP) level and is mediated, at least in part, at the level of transcription of the SLC44A4 gene.
54.2.6.3
Developmental Regulation of the Intestinal Thiamin Uptake Process
The intestinal thiamin uptake process is developmentally regulated during the early stages of life. A decrease in thiamin uptake by mouse intestinal BBMV was observed with maturation (suckling to weanling to adult). This decrease was associated with a decrease in the level of expression of both THTR-1 and THTR-2 ; and a decrease in the activity of the human SLC19A2 and SLC19A3 promoters in the intestine of transgenic mice that carry these promoters. These findings suggest that the developmental regulation of intestinal thiamin uptake is mediated, at least in part, via transcriptional mechanism(s).
54.2.6.4
Differentiation-Dependent Regulation of the Intestinal Thiamin Uptake Process
The intestinal thiamin uptake process also appears to undergo differentiation-dependent regulation. This has been shown in studies utilizing the human intestinal epithelial Caco-2 cells (which differentiate spontaneously in culture upon reaching confluence to become enterocyte-like cells ) and native crypt/villus epithelial cells isolated from wild-type and transgenic mice carrying promoters of the human SLC19A2 and SLC19A3 genes. A significant upregulation in carrier-mediated thiamin uptake by Caco-2 cells was observed as cells differentiate in culture. This upregulation was associated with a significant increase in the level of expression of hTHTR-1 and hTHTR-2 protein and mRNA as well as in activity of the corresponding transfected SLC19A2 and SLC19A3 promoters. Deletion analysis identified the differentiation-responsive region to be at position − 356 to − 275 bp for the SLC19A2 promoter and at position − 77 to − 13 bp for the SLC19A3 promoter. In addition, a critical and specific role in the differentiation-mediated effects for an NF1-binding site (− 348 to − 345 bp) in the SLC19A2 promoter and a SP1/GC-box-binding site (− 48 to − 45 bp) in the SLC19A3 promoter were shown. The physiological relevance of these in vitro findings with Caco-2 cells was confirmed in wild-type and transgenic mice by demonstrating that thiamin uptake and mRNA levels of the mouse THTR-1 and THTR-2, as well as activity of human SLC19A2 and SLC19A3 promoters expressed in transgenic mice, were all significantly higher in intestinal villus compared to crypt epithelial cells. These findings suggest that the differentiation-dependent regulation of intestinal thiamin uptake is, at least in part, mediated via transcriptional mechanism(s).
54.2.6.5
Regulation by Intracellular Signaling Pathways
Studies utilizing human intestinal epithelial Caco-2 cells and the human colonic epithelial NCM460 cells have reported that the intestinal thiamin uptake process is under the regulation of an intracellular Ca 2 + /calmodulin (CaM)-mediated pathway. This pathway appears to act through decreasing the V max (without affecting the apparent K m ) of the intestinal thiamin uptake process, suggesting that the effect is most probably mediated via changes in the activity (and/or number) but not affinity of the thiamin uptake carriers, respectively. The same mode of regulation was also observed for thiamin uptake by other cell types including renal epithelial cells, retinal pigment epithelial cells, and pancreatic beta cells, demonstrating extensive utilization of this regulatory pathway in different tissues.
As to the regulation of the colonic TPP uptake process, this event also appears to be under the regulation of an intracellular Ca + 2 /calmodulin-mediated regulatory pathway.
54.2.7
Conditions/Factors That Negatively Impact the Intestinal Thiamin Uptake Process
54.2.7.1
Effect of Chronic Alcohol Consumption on Intestinal Thiamin Absorption
While chronic alcohol consumption is known to cause impairment in the intestinal thiamin absorption process, which leads to the development of thiamin deficiency in chronic alcoholics, the cellular and molecular mechanisms involved in this impairment have only been recently investigated. A study by Subramanya et al. showed that chronic alcohol feeding of rats leads to inhibition in carrier-mediated thiamin transport across both the brush-border membrane (BBM) and BLM domains of the polarized enterocytes and that the inhibition is evident as early as 2 weeks following the initiation of the alcohol feeding regime. This inhibition was associated with a significant reduction in the expression of THTR-1 (but not THTR-2) at the protein, mRNA, and heterogeneous nuclear RNA (hnRNA) levels (the latter suggests that the effect is, at least in part, mediated at the level of SLC19A2 transcription). Chronic alcohol feeding was also found to inhibit the carrier-mediated thiamin uptake process in the large intestine, suggesting that absorption of the bacterially synthesized thiamin is also a target for inhibition by chronic alcohol consumption. Similarly, chronic alcohol exposure of human intestinal epithelial HuTu-80 cells was found to lead to a significant inhibition in carrier-mediated thiamin uptake, and the effect appears to be, at least in part, mediated at the level of transcription.
54.2.7.2
Effect of Enteropathogens [Enteropathogenic Escherichia coli (EPEC) and Enterotoxigenic Escherichia coli (ETEC)] on Intestinal Thiamin Uptake
Infection with the Gram-negative enteropathogenic Escherichia coli (EPEC), a food-borne pathogen, represents a significant risk to human health. While diarrhea is a major consequence of this infection, malnutrition also occurs especially in severe and prolonged cases, which may aggravate the health status of the infected hosts. A recent study has examined the effect of EPEC infection on thiamin uptake by human intestinal epithelial Caco-2 cells and showed that while wild-type EPEC causes a significant inhibition in thiamin uptake, neither the nonpathogenic E. coli nor killed EPEC, or the filtered supernatant, affect the uptake. The inhibition by EPEC of the intestinal uptake of this B-vitamin does not appear to be a generalized phenomenon affecting intestinal uptake of other water-soluble vitamins, as EPEC did not affect the intestinal uptake of vitamin B 2 (riboflavin) (Ghosal A, Ashokkumar B, and Said HM; unpublished observations). EPEC inhibition of thiamin uptake appears to be mediated via alteration in kinetic parameters of both the nanomolar (mediated by THTR-2) and the micromolar (mediated by THTR-1) saturable thiamin uptake processes. Cell surface expression of the hTHTR-1 and THTR-2 proteins (determined by biotinylation assay) was also significantly decreased in EPEC-treated cells compared to controls. Furthermore, EPEC infection affected the steady-state level of expression of hTHTR-1 and hTHTR-2 mRNA as well as activity of their respective promoters. These studies suggest that EPEC infection has a rapid onset via affecting the expression and activity of the thiamin transporters at the cell membrane, an effect that is then compounded and prolonged by the effects of EPEC on SLC19A2 and SLC19A3 transcription. EPEC structural components needed to cause the inhibition in thiamin uptake were also examined by infecting Caco-2 cells with EPEC mutants that harbor mutations in the escN gene (which encodes a putative ATPase for the EPEC type III secretion system, TTSS), or the espA, espB, or espD genes (which encode structural components of the TTSS) (Ref. and references therein). None of these mutants were found to affect thiamin uptake. On the other hand, mutations in the espF and espH genes (which encode effector proteins) lead to a partial inhibition in thiamin uptake.
Infection with the enterotoxigenic Escherichia coli (ETEC), another enteropathogen that affects a large segment of the global population, also appears to inhibit intestinal thiamin uptake. This was shown recently in studies with Caco-2 monolayers where infection with live ETEC (but not with boiled/killed ETEC or nonpathogenic E. coli ) or treatment with bacterial culture supernatant led to a significant inhibition in thiamin uptake. This inhibition appears to be caused by heat-labile and -secreted ETEC components and is mediated via activation of the epithelial adenylate cyclase system. The inhibition was associated with a significant reduction in expression of human thiamin transporter-1 and -2 (hTHTR1 and hTHTR2) at the protein and mRNA levels as well as in the activity of the SLC19A2 and SLC19A3 promoters. Finally, dual infection of Caco-2 cells with ETEC and EPEC was found to lead to compounded inhibition in intestinal thiamin uptake.
54.2.7.3
Effect of Sepsis on Intestinal Thiamin Uptake
Thiamin deficiency is prevalent in patients with sepsis. This appears to be mediated in part via an effect of sepsis on intestinal thiamin uptake given recently reported observations of a significant inhibition in intestinal thiamin uptake in rat model of sepsis where the degree of inhibition was shown to correlate with the severity of sepsis. Also, the inhibition was associated with a significant decrease in the level of expression of THTR-1 and THTR-2 in the gut mucosa. Sepsis also caused a significant inhibition in level of expression of the mitochondrial TPPT and in level of mucosal ATP.
54.3
Vitamin B 2 (Riboflavin)
54.3.1
Metabolic Role and Effect of Deficiency
Riboflavin (RF; Fig. 54.1 ) is required for normal cellular metabolism, proliferation, and growth. In its biologically active forms [flavin mononucleotide (FMN) and flavin adenine dinucleotide (FAD)], the vitamin plays a key metabolic role in the transfer of electrons in biological oxidation-reduction reactions involving carbohydrate, lipid, amino acid, and certain water-soluble vitamins (pyridoxine and folate). Studies have also shown a role for riboflavin in protein folding in the endoplasmic reticulum and in the regulation of cellular energy expenditure. More recent investigations have demonstrated antioxidant and antiinflammatory properties for riboflavin, as well as a role for this vitamin in normal immune function. Riboflavin deficiency leads to a variety of clinical abnormalities, including degenerative changes of the nervous system, anemia, skin lesions, cataract, and growth retardation. It also leads to an increase in the susceptibility to cancer. Deficiency/suboptimal levels of riboflavin occur in chronic alcoholism, diabetes mellitus, IBD, Brown-Vialetto Van Laere, and Fazio Londe syndromes [the latter are rare neurological disorders caused by mutations in riboflavin transporters-2 and -3 (RFVT-2 and RFVT-3)]. In contrast to the deleterious effect of riboflavin deficiency, optimizing riboflavin status has been reported to reduce the risk of esophageal squamous cell carcinoma (Ref. and references therein).
54.3.2
Physiology of the Intestinal Riboflavin Absorption Process
The human intestine encounters two sources of RF: a dietary source and a microbiota source. Dietary riboflavin exists mainly in the form of FMN and FAD and is bound (noncovalently) to proteins. These coenzymes are first released from proteins via the combined action of gastric acid and hydrolases and then hydrolyzed further (prior to absorption) by intestinal phosphatases to free riboflavin. The mechanism of absorption of free riboflavin has been studied using a variety of human and animal intestinal preparations. Collectively, these studies have shown that transport of free riboflavin occurs mainly in the proximal part of the small intestine and involves an efficient and specific Na + -independent, carrier-mediated mechanism. This mechanism is inhibited by riboflavin structural analogues and by the Na + /H + exchange inhibitor amiloride ( K i for amiloride ≈ 0.48 mM). Other studies have shown that the intestinal riboflavin uptake process is sensitive to the effect of the tricyclic phenothiazine drug chlorpromazine, a compound that shares structural similarities with riboflavin. Once internalized, some of the riboflavin is phosphorylated and used by enterocytes, and the rest is transported out of the cell across the BLM via a specific carrier-mediated process.
With regard to the microbiota-generated riboflavin in the large intestine, the amount of the vitamin produced depends on the type of the ingested diet and is higher following ingestion of a vegetable-based diet compared to a meat-based diet. Also, a considerable amount of the microbiota produced riboflavin was found to exist in the large intestinal lumen in the form of free riboflavin, and thus, available for absorption. Other investigations have shown that the large intestine is capable of absorbing luminally introduced free riboflavin. Insight into the mechanism involved in colonic uptake of riboflavin came from studies using the human colonic epithelial NCM460 cells, which showed the involvement of an efficient and specific carrier-mediated mechanism that is similar to the one described in the small intestine. Subsequent studies in rats have confirmed the existence of a specialized carrier-mediated mechanism for riboflavin uptake in the colon. With the demonstration of existence of an efficient carrier-mediated system for riboflavin uptake in the large intestine, the belief that this source of riboflavin contributes to the overall host nutrition of the vitamin, and especially toward the cellular nutrition of the localized colonocytes, is further strengthened.
54.3.3
Molecular Biology of the Intestinal Riboflavin Uptake Process
Insight into the molecular identity of the transport systems involved in intestinal riboflavin uptake has recently emerged with the identification of three putative human riboflavin transporters-1, -2, and -3 (RFVT-1, RFVT-2, and RFVT-3) that are encoded by three different but homologous genes: the SLC52A1 , SLC52A2 , and SLC52A3 genes, respectively. These transporters exhibit no similarity with the yeast or bacterial riboflavin transporters, nor do they show similarity with any other mammalian transporter. The hRFVT-1 and hRFVT-2 share > 87% sequence similarity at the protein level, while hRFVT-3 shares around 43% similarity with hRFVT-1 and hRFVT-2. The hRFVT-1, -2, and -3 are all expressed in the gut, with expression of the hRFVT-3 being significantly higher than that of hRFVT-1 and hRFVT-2 ; hRFVT-3 is also more efficient in transporting riboflavin than the other hRFVTs. Furthermore, live-cell confocal imaging of polarized intestinal (and renal) epithelial cells showed the hRFVT-3 to be predominantly expressed at the apical membrane domain, while expression of hRFVT-1 is mostly at the BLM domain of these cells; expression of hRFVT-2 was localized inside intracellular vesicles (with some expression at the BLM domain).
The relative contribution of the predominantly expressed riboflavin transporter in the intestine (i.e., RFVT-3) toward total carrier-mediated riboflavin uptake has also been recently addressed using an in vitro SLC52A3 gene-specific silencing siRNA approach and human intestinal epithelial Caco-2 cells with the results showing a major role for these transporters in intestinal riboflavin uptake. This was confirmed in vivo in studies utilizing an intestinal-specific (conditional) RFVT-3-knockout (cKO) mouse model developed by the Cre/Lox approach ( Fig. 54.7 ). In the latter study, all the RFVT-3 cKO mice developed systemic riboflavin deficiency indicating that RFVT-3 is involved in determining the overall riboflavin body homeostasis. Also observed was a significant increase in the level of expression of oxidative stress-responsive genes in the intestine of the cKO mice, which suggest a role for riboflavin in the maintenance of normal intestinal physiology.

54.3.4
Cell biology of the Intestinal Riboflavin Absorption Process
As mentioned earlier, the RFVT-3 is expressed exclusively at the apical membrane domain of intestinal epithelial cells. What dictates cell surface expression of the protein appears to be a sequence in the C-terminal portion of the polypeptide that includes two conserved cysteine residues (one at position 463 and the other at position 467). Mutating these cysteine residues was shown to lead to severe impairment in riboflavin uptake due to retention of the protein in the endoplasmic reticulum. A potential disulfide bridge between C463 and C386 (also predicted by modeling analysis and mutating the C386 residue) was found to lead to intracellular retention of RFVT-3. Intracellular trafficking of RFVT-3 was shown to involve distinct vesicular structures whose mobility depends on existence of an intact microtubule network.
54.3.5
Structure-Activity Aspects of the Riboflavin Transporters
Early studies using Caco-2 cells exposed to specific sulfhydryl group reagents have reported a role for sulfhydryl groups in the function of the intestinal riboflavin uptake carriers. Since the sulfhydryl group reagents used in these studies were membrane impermeable, it was assumed that the affected sulfhydryl group(s) were located at the exofacial surface of the cell membrane. More recent investigations examined the effect of clinical mutations in SLC52A3 found in patients with the Brown-Vialetto-Van Laere syndrome (BVVLS; a rare neurological disease characterized by ponto-bulbar palsy, bilateral sensorineural deafness, and respiratory insufficiency) on cell biology of RFVT-3. Mutants P28T, E36K, E71K, and R132W were all found to be functionally impaired and that the impairment was due to retention of the mutated RFVT-3 within the endoplasmic reticulum. These findings suggest a role for the affected residues in normal cell biology of RFVT-3.
54.3.6
Regulation of the Intestinal Riboflavin Uptake Process
The intestinal riboflavin uptake process is under the regulation of extracellular/environmental conditions and intracellular factors. Since a number of these conditions exert their effect(s) at the transcription level, it is relevant to first describe the basal transcriptional regulation of the intestinally relevant SLC52A1 and SLC52A3 genes.
54.3.6.1
Transcriptional Regulation of the SLC52A1 and SLC52A3 Genes
Recent studies have reported the cloning of the 5′-flanking region of the SLC52A1 gene and its characterization in human intestinal epithelial cells. These studies showed the gene to have one transcription start site (TSS) and has a core (minimal) promoter between − 234 and − 23. This minimal promoter lacks TATA elements, but is GC-rich and harbors several putative cis -regulatory (SP-1, KLFs, AP-2, and EGRF), of which Sp-1 appears to be the most relevant. Promoter supershift and chromatin immunoprecipitation (ChIP) analyses have confirmed the interaction between the Sp-1 cis-element and its Sp-1 nuclear factor; also an enhancement in promoter activity was observed following cotransfection of the minimal SLC52A1 promoter with Sp-1 into Drosophila SL-2 cells (which lacks endogenous Sp activity).
Identification of the minimal 5′-regulatory region of the SLC52A3 gene and determination of the regulatory element(s) involved in its activity in intestinal epithelial cells, together with confirmation of promoter activity in vivo in transgenic mice (to establish physiological relevance) have recently been accomplished. The minimal SLC52A3 promoter was found to be located between − 199 and + 8 bp (using the start of the TSS as position 1). An important role for the Sp1-binding site (at position − 74/−71 bp) in determining the activity of the SLC52A3 promoter was also identified. The latter has been established by means of mutational analysis, binding of Sp1 to the minimal SLC52A3 promoter (i.e., EMSA and supershift assays), and by ChIP analysis. The importance of Sp1 in driving the activity of the SLC52A3 minimal promoter was further confirmed in studies utilizing Drosophila SL-2 cells (which lack Sp activity) where a significant induction in the activity of the SLC52A3 minimal promoter upon cotransfection with the Sp3 nuclear factor was observed. Activity of the cloned SLC52A3 promoter was further confirmed in vivo in transgenic mice carrying the promoter construct fused to luciferase.
54.3.6.2
Adaptive Regulation of the Intestinal Riboflavin Uptake Process
The intestinal riboflavin uptake process is adaptively regulated by the prevailing extracellular substrate level. This has been shown in vivo in rats supplemented with different levels of riboflavin and in vitro in studies utilizing cultured intestinal epithelial cells. In the rat study, induction of riboflavin deficiency (by dietary means) led to a significant and specific upregulation in intestinal riboflavin uptake, while oversupplementation of the animals with riboflavin led to a significant and specific downregulation in intestinal riboflavin uptake. In the in vitro studies, maintaining human intestinal epithelial cells (Caco-2 and NCM460) in a RF-deficient medium also led to a significant upregulation in riboflavin uptake, while maintaining these cells in the presence of high pharmacological concentrations of the vitamin led to downregulation in riboflavin uptake. The latter adaptive changes were mediated via changes in the V max (but not the apparent K m ) of the riboflavin uptake process, suggesting that the changes are mediated via an increase in the number (and/or activity) but not the affinity of the riboflavin carriers. Actinomycin D treatment of cells led to significant inhibition in the induction of riboflavin uptake under riboflavin deficiency, thus suggesting possible involvement of a transcriptional mechanism(s) in the adaptive response. The latter suggestion was confirmed in recent investigations showing the induction in riboflavin uptake in riboflavin deficiency to be associated with an increase in expression of the hRFVT-3 (and hRFVT-2; but not hRFVT-1). Focusing predominantly on the hRFVT-3, studies showed an induction in the level of expression of the hRFVT-3 protein, mRNA, and hnRNA, as well as in activity of its gene ( SLC52A3 ) promoter in deficiency. The latter studies also showed an increase in the level of expression of the Sp1 nuclear factor (which as mentioned above is important for activity of the SLC52A3 promoter) in riboflavin deficiency, and that mutating the Sp1/GC site in the SLC52A3 promoter led to a drastic decrease in the level of induction in SLC52A3 promoter activity in riboflavin deficiency. Furthermore, the study reported involvement of specific epigenetic alterations affecting the SLC52A3 promoter in riboflavin deficiency, and an increase in hRFVT-3 protein expression at the cell surface under the deficiency condition.
54.3.6.3
Developmental Regulation of the Intestinal Riboflavin Uptake Process
Whether the intestinal riboflavin uptake process is developmentally regulated has also been addressed. Riboflavin transport in rat intestine declines with maturation; this decline is mediated via a decrease in the V max and an increase in the apparent K m of the carrier-mediated riboflavin uptake process (i.e., as the animal moves from suckling to weanling to adulthood). The latter findings suggest that developmental maturation of the intestine is associated with a decrease in the number (and/or activity) of the riboflavin uptake carriers and a decrease in their affinity. The molecular mechanism(s) involved in the developmental regulation of intestinal riboflavin uptake, however, is not currently known and in need of further investigations.
54.3.6.4
Differentiation-Dependent Regulation of Intestinal Riboflavin Uptake Process
The intestinal riboflavin uptake process also appears to undergo differentiation-dependent regulation. This was shown in studies utilizing, as models, the human-derived intestinal epithelial Caco-2 cells and native rat intestine. Carrier-mediated riboflavin uptake was found to be significantly higher in postcompared to preconfluent Caco-2 cells. This was found to be associated with a significantly higher level of expression of hRFVT-1 and hRFVT-3 protein and mRNA in the postcompared to the preconfluent cells ; it was also accompanied with a significantly higher rate of transcription of the SLC52A1 and SLC52A3 genes as indicated by the higher level of expression of their hnRNA and higher activity of their promoters in postcompared preconfluent Caco-2 cells. Studies with native rat intestine showed similar results in that a significantly higher riboflavin uptake was found in epithelial cells of the villus tip compared the crypt, which was associated with a significantly higher level of expression of the rat RFVT-1 and RFVT-3 protein, mRNA, and hnRNA. Collectively, these findings suggest that the differentiation-dependent regulation in intestinal riboflavin uptake is mediated (at least in part) via transcriptional mechanism(s).
54.3.6.5
Regulation by Intracellular Signaling Pathways
Evidence has been presented to suggest that the intestinal riboflavin uptake process is under the regulation of an intracellular protein kinase-A (PKA)-mediated signaling pathway. Activation of this signaling pathway was found to lead to a significant inhibition in riboflavin uptake by the human intestinal epithelial Caco-2 cells. This inhibition was reversible and appears to be mediated via a significant decrease in the activity (and/or the number) of the riboflavin uptake carriers with no change in their affinity. Other findings (using cycloheximide, actinomycin D, colchicine, and cytochalasin D) have suggested that the PKA-mediated inhibition of riboflavin uptake by Caco-2 cells was not mediated via inhibition of the synthesis of the riboflavin carrier protein(s) or inhibition in the recruitment of preexisting carrier protein(s) into the plasma membrane. The intestinal riboflavin uptake process also appears to be under the regulation of another intracellular regulatory pathway, namely, the Ca 2 + /calmodulin-mediated signaling pathway. Regulation by this pathway appears to be mediated via changes in both the activity (and/or number) and affinity of the involved riboflavin uptake carriers.
54.3.7
Factors and Conditions That Negatively Impact the Intestinal Riboflavin Uptake Process
Chronic alcohol consumption in humans is associated with a higher prevalence of riboflavin suboptimal/deficient levels. This appears to be mediated, at least in part, via interference with intestinal riboflavin absorption process as shown in studies utilizing rats chronically fed an alcohol liquid diet. These studies showed that chronic alcohol feeding leads to a significant inhibition in carrier-mediated riboflavin uptake across both the jejunal brush-border and BLM. This inhibition was found to be associated with a parallel reduction in the expression of RFVT-1 and RFVT-3 at the protein, mRNA, and hnRNA levels. The latter suggest that the inhibitory effect of chronic alcohol feeding is mediated, at least in part, at the level of transcription of the slc52a1 and slc52a3 genes. Chronic alcohol feeding was also shown to inhibit colonic uptake of riboflavin. Thus, absorption of both dietary- and microbiota-generated riboflavin appears to be negatively impacted by chronic alcohol exposure.
Other studies have shown that the Na + /H + exchanger amiloride and the tricyclic phenothiazine agent, chlorpromazine (which shares structural similarities with RF), inhibit intestinal riboflavin uptake.
54.4
Vitamin B 3 (Niacin, Nicotinic Acid)
54.4.1
Metabolic Role and Effect of Deficiency
The main function of niacin ( Fig. 54.1 ) is as a precursor for the synthesis of the coenzymes nicotinamide adenine dinucleotide (NAD) and nicotinamide adenine dinucleotide phosphate (NADP). Most of the NAD- and NADP-linked enzymes are involved in reactions that maintain the redox state of cells such as glycolysis and the pentose phosphate shunt. Niacin also appears to have lipid-lowering effects and is in use clinically for that purpose. Severe niacin deficiency in humans leads to pellagra, a disease characterized by inflammation of mucous membranes, skin lesions, and diarrhea. Deficiency and suboptimal levels of niacin occurin chronic alcoholism and in patients with Hartnup’s disease. Patients with the latter disorder have mutations in the gene that encodes the transporter of the amino acid tryptophan, which is a precursor for the endogenous production of niacin.
54.4.2
Physiology of the Intestinal Niacin Absorption Process
Humans have access to niacin from endogenous and exogenous sources. The endogenous source of niacin is provided via the metabolic conversion of tryptophan to niacin via the so called “kunurenine pathway.” The exogenous source is provided through the diet and via production of the vitamin by the large intestinal microbiota. Our knowledge of the mechanism of intestinal absorption of dietary niacin has expanded in recent years. Early animal studies have concluded that niacin is taken up by intestinal absorptive cells either via simple diffusion of the undissociated form of nicotinic acid (i.e., according to the pH partition hypothesis and is assisted by acidic microclimate at the luminal surface of the intestine; p K a of nicotinic acid is 4.9), or via a carrier-mediated mechanism involving a monocarboxylate transporter(s). The latter studies, however, have reported an apparent K m of 3.52–17.0 mM. Such a high apparent K m raises a concern with regard to the physiological relevance of the described system, because intestinal luminal concentration of niacin under physiological conditions is estimated to be in the micromolar range. Recent studies, however, using human intestinal epithelial Caco-2 cells and purified intestinal BBMV isolated from human organ donors have shown the involvement of an acidic pH-dependent (but Na + -independent) high-affinity carrier-mediated mechanism for niacin uptake (apparent K m of 0.53 μM). This system is highly specific for niacin and is not affected by monocarboxylic acids. In addition, the system was highly sensitive to the effect of membrane-impermeable sulfhydryl group reagents and appears to be under the regulation of an intracellular protein tyrosine kinase (PTK)-mediated signaling pathway. A similar high affinity carrier-mediated system (apparent K m of 0.73 ± 0.16 μM) was subsequently described for niacin uptake in human liver cells. Currently, there is nothing known about the molecular identity of the intestinal niacin uptake system or about how the vitamin exits the polarized enterocytes across the BLM.
As to the microbiota-generated niacin in the large intestine, recent studies utilizing human colonic epithelial NCM460 cells, native human colonic apical membrane vesicles (AMV) isolated from organ donors, and mouse colonic loops in vivo as models have shown the existence of an acid pH (but not Na + dependent) carrier-mediated (apparent K m of 2.5 μM) process for niacin uptake. The process was also shown to be probenecid sensitive, but was not affected by other bacterially produced monocarboxylates. Furthermore, the colonic niacin uptake process was found to be under the regulation of an intracellular PTK- and a Ca 2 + -calmodulin-mediated regulatory pathways; it was also adaptively regulated by the prevailing substrate level in the extracellular environment.
54.5
Vitamin B5 (Pantothenic Acid)
54.5.1
Metabolic Role and Effect of Deficiency
Pantothenic acid ( Fig. 54.1 ) is required for the biosynthesis of coenzyme A and acyl carrier proteins in mammalian tissues, and thus is involved in the metabolism of carbohydrate, fat, and to a lesser extent protein. Spontaneous pantothenic acid deficiency has not been reported in humans, most likely because of the ubiquitous distribution of the vitamin.
54.5.2
Physiology of the Intestinal Pantothenic Acid Absorption Process
The intestinal tract is exposed to two sources of pantothenic acid, one being dietary and the other is bacterial (i.e., microbiota-generated vitamin; Ref. ). Dietary pantothenate exists mainly in the form of coenzyme A, which is hydrolyzed to free pantothenic acid in the intestinal lumen prior to uptake. Free pantothenic acid is then transported into the absorptive cells via the sodium-dependent multivitamin transporter, SMVT, which also transports biotin and lipoate (Refs. ; see also Section 54.7 ). Absorption of the microbiota-generated pantothenic acid in the large intestine also appears to utilize the SMVT system. There is little known about regulation of the intestinal pantothenic uptake process, but it may be similar to the regulation of intestinal biotin uptake process since the two vitamins share the same intestinal uptake system. There is nothing known at present regarding the mechanism of exit of pantothenic acid out of the intestinal absorptive cells.
54.6
Vitamin B 6 (Pyridoxine and Derivatives)
54.6.1
Metabolic Role and Effect of Deficiency
Pyridoxine ( Fig. 54.1 ) and other members of the B 6 family (pyridoxal and pyridoxamine) exist in varying proportions in the diet in both the phosphorylated and nonphosphorylated forms. Pyridoxal 5′-phosphate represents the most biologically active form of the vitamin and is a cofactor for enzymes that catalyze transaminase, decarboxylase, and synthetase reactions in pathways that include carbohydrate, lipid, protein (including metabolism of the amino acid homocysteine), and in production of neurotransmitters. Vitamin B 6 deficiency in humans leads to a variety of clinical abnormalities that includes microcytic anemia, dermatitis/glossitis, and neurological disorders. Deficiency/suboptimal levels of vitamin B 6 occur in conditions such as chronic alcoholism and in diabetes, in patients with celiac and renal diseases, and following long-term use of hydrazines (e.g., isoniazid, which is used for treatment of tuberculosis) as well as penicillamine. Patients with vitamin B 6 -dependent seizure, an autosomal-recessive disorder (believed to be due to an abnormality in pyridoxine transport into cells), also shows low blood levels of vitamin B 6.
54.6.2
Physiology of Intestinal Vitamin B 6 Transport
Vitamin B 6 is synthesized by plant cells and by most unicellular microorganisms. However, humans and other mammals cannot synthesize the vitamin, and thus must obtain the micronutrient from exogenous sources via intestinal absorption. As with a number of other water-soluble vitamins, two sources of vitamin B 6 are available to the intestine: a dietary source and a bacterial source (the latter is in references to the vitamin generated by the microbiota of the large intestine; Ref. ). Dietary phosphorylated forms of vitamin B 6 are hydrolyzed to free forms prior to absorption. A variety of intestinal preparations have been used to study the mechanisms(s) of intestinal absorption of vitamin B 6 . Some studies have reported inability of the intestine to accumulate vitamin B 6, while others have reported lack of a saturable uptake process. This led to the conclusion that intestinal vitamin B 6 uptake is via simple diffusion process. This conclusion, however, has been challenged by Said et al. who used human intestinal epithelial Caco-2 cells to show the existence of an efficient and specific carrier-mediated mechanism for pyridoxine uptake. The latter studies have also shown that the intestinal uptake process of pyridoxine is highly dependent on acidic extracellular pH, but is Na + -independent. In addition, amiloride was found to inhibit (in a competitive manner) pyridoxine uptake by intestinal epithelial cells (apparent K i of 0.39 mM). These findings on the involvement of carrier-mediated pyridoxine uptake process in intestinal epithelial cells are similar to those reported for the vitamin uptake by renal epithelial cells. Other recent investigations have reported an important role for the intestine in vitamin B 6 metabolism.
With regard to the microbiota-generated vitamin B 6 , a significant amount of this source of vitamin exists in the free form in the surrounding medium (i.e., not trapped inside bacterial cells), and thus is available for absorption. Evidence suggesting that this source of vitamin B 6 is indeed bioavailable to the host comes from studies showing that the amount of vitamin B 6 excreted in the urine is significantly higher than the total amount of the vitamin consumed orally. Direct evidence for the ability of the colonic epithelial cells to absorb vitamin B 6 came from studies using mouse and human colonic preparations, which showed the involvement of an efficient and specific carrier-mediated mechanism (apparent K m of 2.1 μM) for pyridoxine uptake.
Currently, there is little known about the molecular identity of the intestinal pyridoxine carrier in any species. However, the structure of the first vitamin B 6 transporter in any living organism ( Saccharomyces cerevisiae ) has been reported. This system was predicted to have 12 TMDs and appears to be regulated by substrate availability in the extracellular environment.
54.6.3
Regulation of the Intestinal Vitamin B 6 Uptake Process
The intestinal vitamin B 6 uptake process appears to be under the regulation of intracellular and extracellular factors. A role for a PKA-mediated signaling pathway in regulating intestinal pyridoxine uptake has been reported. In the latter study, pretreatment of Caco-2 cells with compounds that increase in intracellular cAMP levels, and thus activate PKA, caused a significant inhibition in pyridoxine uptake. This inhibition was mediated via a decrease in the activity (and/or the number), but not affinity, of the pyridoxine uptake carriers. The molecular mechanism through which cAMP exerts its effect on intestinal pyridoxine uptake process, however, is not clear and requires further investigations.
The intestinal pyridoxine uptake process is also adaptively regulated by the prevailing extracellular pyridoxine levels, with a significant induction in the uptake occurring in cells maintained in the presence of low pyridoxine level compared to those maintained in the presence of high level. The mechanism involved in this adaptive regulation appears to be, at least in part, mediated via transcriptional mechanism(s).
54.7
Vitamin B 7 (Biotin)
54.7.1
Metabolic Role and Effect of Deficiency
Biotin ( Fig. 54.1 ) is an indispensable micronutrient for normal human health due to its essential nature for cellular metabolism, proliferation, and survival. Biotin acts as a cofactor for five carboxylases that are critical for fatty acid, glucose, and amino acid metabolism. Important roles for biotin in cellular energy metabolism (i.e., ATP production) and in regulation of cellular oxidative stress, as well as in gene expression (where expression of over 2000 human genes appears to be affected by biotin status ) have also been reported. Emerging evidence has also been accumulating documenting an important role for biotin in the function of the immune system. In reference to the latter, biotin was shown to be important for activity of the human dendritic cells and the human natural killer (NK) lymphocytes, for the generation of cytotoxic T lymphocytes, and for the maturation and responsiveness of immune cells. An increase in the levels of proinflammatory cytokines such as tumor necrosis factor-α (TNF-α), interleukin-1b, and interferon-γ (IFN-γ) has been observed in biotin deficiency. Biotin also appears to play an important role in the maintenance of normal intestinal integrity and homeostasis as its deficiency leads to an increase in intestinal permeability and changes in level of expression of tight junction (TJ) proteins, as well as to spontaneous inflammation in the large intestine. Biotin also appears to influence the colonization and invasiveness of certain enteropathogenic bacteria, and it mediates the effect of probiotic bacteria on microbial community in the gut. Finally, animal studies have shown that biotin deficiency during pregnancy could lead to embryonic growth retardation, congenital malformation, and death. Thus, it is not surprising that deficiency/suboptimal levels of this vitamin lead to disturbances in the normal function of many organ systems and ultimately to clinical manifestations of disease. The latter includes dermatological abnormalities, neurological disorders, and growth retardation (reviewed in Refs. ). Deficiency/suboptimal levels of biotin occur in conditions including IBD, chronic alcoholism, in patients on long-term therapy with anticonvulsant drugs, and those receiving long-term parenteral nutrition. In addition, inborn errors in biotin metabolism (multiple carboxylase deficiency ) and patients with biotin-responsive basal ganglia disease (an autosomal recessive disease) appear to benefit from biotin supplementation implying suboptimal cellular levels of the vitamin.
54.7.2
Physiology of the Intestinal Biotin Absorption Processes
The human intestine is exposed to two sources of biotin: a dietary source and a bacterial source (the latter is in reference to the biotin that is generated by the gut microbiota). Dietary biotin exists both in free and protein-bound forms. The protein-bound form is first digested to biocytin (biotinyl- l -lysine) and biotin-short peptides via the action of gastrointestinal (GI) proteases and peptidases. Biocytin and biotin-short peptides cannot be absorbed as such (cannot be recognized by the intestinal biotin uptake system) until they are further digested into free biotin, a process performed by biotinidase, an enzyme that is believed to be of pancreatic origin. Biotinidase has been cloned, and a number of clinical mutations have been identified in its sequence in patients with the autosomal recessive disorder “biotinidase deficiency.” Patients with the latter disorder display neurological and cutaneous abnormalities. These patients cannot convert biocytine or biotin-short peptides to free biotin in the intestinal lumen nor can they recycle endogenous biocytin (and biotin-short peptides) that is generated from catabolism of biotin-bound enzymes/proteins to free biotin in different tissues. This leads to a reduction in the fraction of dietary biotin that is bioavailable/absorbed, and to a reduction in ability of different cells to take in sufficient amounts of this vitamin. Supplementation with high pharmacological doses of “free” biotin brings about a favorable clinical outcome in these patients as; such a treatment could prevent the development of the abnormal clinical symptoms if initiated early.
Absorption of free biotin (a substrate that is negatively charged at physiological pH) in the small intestine occurs via an efficient Na + -dependent carrier-mediated mechanism; absorption is higher in the proximal compared to the distal part of the small intestine. Functional, immunological, and live-cell confocal imaging studies have localized the Na + -dependent, carrier-mediated, biotin uptake system at the apical BBM domain of the polarized enterocytes. The exit of biotin from the polarized absorptive cells across the BLM is also carrier-mediated, but is Na + -independent in nature. One of the interesting features of the intestinal Na + -dependent biotin absorption process is its ability to transport not only free biotin but also two other functionally unrelated nutrients, namely, the water-soluble vitamin pantothenic acid (which is important for energy, fat, and protein metabolism) and the metabolically important substrate, lipoate (a potent intracellular and extracellular antioxidant). It is for the latter reason that the uptake system involved in the uptake of biotin is referred to as the “sodium-dependent multivitamin transporter,” or “SMVT.”
With regard to the biotin generated by the gut microbiota, a substantial portion of this biotin exists in the free form, and thus available for absorption. While gut microbiota of all humans generates biotin, recent studies that classified the human gut microbiome into three functional enterotypes of which enterotype 1 is especially overrepresented in enzymes involved in the biotin biosynthetic pathway, therefore, implying a larger level of biotin generation in the group that harbors this enterotype. As to absorption of the microbiota-generated biotin, both human and animal colonocytes are capable of absorbing the vitamin via the same SMVT-dependent process that operates in the small intestine. The demonstration of the existence of a carrier-mediated mechanism for absorption of the microbiota-generated biotin in the large intestine clearly suggests that this source of biotin is bioavailable and contributes toward overall host biotin nutrition, and especially toward the cellular nutrition of the local colonocytes. The latter may have consequences in relation to the maintenance of normal colonic health.
54.7.3
Molecular Biology of the Intestinal Biotin Uptake Process
The SMVT system (product of the SLC5A6 gene) has been cloned from the intestine of a number of species including human. Functional identity of the cloned SMVT was established by expression in a number of heterologous cellular systems, where the protein was found to transport both biotin and pantothenic acid in a Na + -dependent manner and with similar kinetic parameters as those determined in native intestinal preparations (reviewed in Ref. ). Functional, immunological, and confocal imaging studies have shown that the protein is expressed exclusively at the apical membrane domain of the polarized intestinal epithelial cells ( Fig. 54.8 ). The SMVT polypeptide is predicted to have 12 TMD and is N-glycosylated at N138 and N489. This glycosylation appears to be important for function of the SMVT system. In addition, putative phosphorylation sites for several intracellular protein kinases are predicted in the SMVT polypeptide; of these putative sites, the T286 site has been experimentally shown to be important for the protein kinase-C (PKC)-mediated regulation of biotin uptake.

While another potential biotin transport system has been suggested in other tissues, the SMVT system appears to be the only system that operates in the mammalian intestinal tract. The latter conclusion is based on recent findings from in vitro studies utilizing gene-specific silencing (siRNA) approach, and in vivo investigations utilizing conditional (intestinal-specific) SMVT-knockout (cKO) mouse model, which is generated by utilizing Cre/lox technology. In the latter studies, a complete inhibition of gut biotin (and pantothenic acid) uptake in the SMVT-cKO mice was observed. Also, all the cKO animals developed biotin deficiency, severe growth retardation, and decreased bone density and length. Furthermore, two-third of the cKO animals died prematurely prior to reaching the age of 10 weeks. An unexpected observation found in all the SMVT-cKO animals was the development of a spontaneous intestinal chronic active inflammation, especially in the cecum ( Fig. 54.9 ). This inflammation is reminiscent to that seen in IBD. The latter finding suggests that the SMVT system plays a role in the maintenance of normal intestinal mucosal integrity and immunity. The suggestion was confirmed by subsequent investigations showing a significant increase in gut permeability and marked changes in level of expression of TJ proteins in the intestine of the SMVT-cKO mice ( Fig. 54.10 ). These changes were found to be related to the state of biotin deficiency that develops in the SMVT-cKO mice, as shown in studies utilizing wild-type mice made biotin deficient by dietary means. In the latter studies, chronic active inflammation also developed in the cecum of the biotin-deficient mice compared to their pair-fed controls ( Fig. 54.11 ); and was associated with a significant increase in the level of expression of proinflammatory cytokines in the cecal mucosa, an increase in intestinal permeability, and changes in level of expression of TJ proteins ( Fig. 54.12 ). Since no changes in permeability and expression of TJ proteins occur in confluent intestinal epithelial Caco-2 monolayers maintained under chronic biotin-deficient conditions, it was suggested that the role of intestinal SMVT in the maintenance of normal mucosal integrity is most likely mediated via its function in providing biotin to immune cells of the gut mucosa. The latter is likely given the roles played by biotin in maintaining normal innate and adaptive immune functions.




54.7.4
Cell Biology of the Intestinal Biotin Absorption Process
As mentioned earlier, the SMVT protein is expressed exclusively at the apical membrane domain of intestinal epithelial cells ( Fig. 54.8 ). What determines targeting of the hSMVT protein to the BBM of these cells has been investigated using live-cell confocal imaging and shown to be dictated by a sequence(s) in the C-terminal tail of the polypeptide. Furthermore, distinct trafficking vesicles were found to be involved in the intracellular movement of SMVT, and that mobility of these vesicles appears to be dependent on the existence of intact microtubule network.
The SMVT protein also appears to have an interacting partner, the PDZ domain containing 11 (PDZD11) in intestinal epithelial cells that affects its function ( Fig. 54.4 ). The interaction between SMVT and its partner was shown in studies utilizing yeast two-hybrid screening approach, and was confirmed by in vitro (by GST-pull-down assay), in vivo (by two-hybrid luciferase and coimmunoprecipitation assays), and confocal imaging of living cells. Functional consequence of this interaction was shown in studies involving coexpression of the SMVT with PDZD11, which led to an increase in biotin uptake; on the other hand, knocking down PDZD11 was found to lead to an inhibition in the vitamin uptake. Finally, the interaction of PDZD11 with the SMVT polypeptide was found to occur at the PDZ-binding domain of the transporter located at its cytoplasmic tail.
54.7.5
Structure-Activity Aspects of the hSMVT
Knowledge about the structure – function relationship of the hSMVT has also been forthcoming in recent years. In one study, the role for each of the 10 conserved (among species) cysteine residues in the function of hSMVT has been examined following their individual mutation by mean of site-directed mutagenesis. Only C294 was found to be essential for functionality, and its role appears to be mediated via insuring a proper expression of the hSMVT protein at the cell surface as shown by biotinylation assay.
Other studies have shown that the hSMVT polypeptide is glycosylated at positions N138 and N489 and that this glycosylation is important for its function.
54.7.6
Regulation of Intestinal Biotin Absorption Process
A variety of extracellular/environmental conditions and intracellular factors appear to regulate the intestinal biotin uptake process. Since a number of these conditions/factors work via transcriptional mechanism(s), it is relevant to first describe the basic transcriptional regulation of the SLC5A6 gene (which encodes SMVT) in human intestinal epithelial cells. In this regard, the 5′-regulatory region (promoter) of the human SLC5A6 genes was found to have two promoters (promoters 1 and 2). Activity of these promoters was confirmed in vitro using the Firefly luciferase reporter gene assay following transient transfection into human intestinal epithelial cells, and in vivo in transgenic mice carrying the hSMVT promoter-luciferase construct. A role for the nuclear factors Kruppel-like factor 4 (KLF-4) and activator protein (AP-2) in regulating the activity of the SLC5A6 promoter has also been reported.
54.7.6.1
Adaptive Regulation of the Intestinal Biotin Uptake Process
The small intestinal and colonic biotin uptake processes are adaptively regulated by extracellular biotin level. Biotin deficiency leads to a specific and significant upregulation in intestinal carrier-mediated biotin uptake, while oversupplementation with high pharmacological concentrations of biotin leads to a specific and significant downregulation in uptake. This adaptive regulation in biotin uptake is associated with changes in level of expression of SMVT protein and mRNA. Thus, in biotin deficiency, a significant and specific upregulation in carrier-mediated biotin uptake associated with induction in the level of expression of SMVT protein and mRNA were observed. The increase in SMVT mRNA level in deficiency is not due to an increase in RNA stability but rather due to an increase in the activity of the SLC5A6 promoter. The biotin deficiency-responsive region in the SLC5A6 promoter has also been mapped and shown to be in a 103-bp region that contains gut-enriched Kruppel-like factor (GKLF) cis-elements that confer the upregulatory response to biotin deficiency.
54.7.6.2
Developmental Regulation of the Intestinal Biotin Uptake Process
The intestinal biotin uptake process undergoes developmental regulation during early stage of life. Studies in rats have shown that the preferential site of biotin absorption during the suckling period is the ileum, which changes to the jejunum in adulthood. The observed change in the jejunum was mediated via a progressive increase in V max of the biotin carrier-mediated uptake process as the animals move from suckling to weanling to adulthood. These changes are associated with a parallel increase in the level of expression of intestinal SMVT protein and mRNA and in the transcription activity of the Slc5a6 gene.
54.7.6.3
Regulation by Intracellular Signaling Pathways
PKC-mediated pathway also appears to play a role in regulating the intestinal biotin uptake process. This regulation is mediated via a decrease in the V max of the uptake process, suggesting changes in the activity (and/or number) of the carrier system. PKC appeared to exert its regulatory effect on biotin uptake via T286 of the SMVT polypeptide, as mutation of this site leads to a significant decrease in PKC regulatory effect on hSMVT function.
A role for casein kinase-2 (CK-2) in regulating surface expression of SMVT in colonic epithelial cells (and thus regulating rate of biotin uptake) has also been recently described (Lakhan R and Said HM; manuscript in preparation). This effect appears to be mediated via the CK-2 phosphorylation site located at position 78 of the SMVT polypeptide.
54.7.7
Factors That Affect the Intestinal Biotin Uptake Process
A significant reduction in plasma biotin levels has been observed in chronic alcoholics. Studies utilizing animal models that were fed alcohol chronically have shown that this, at least in part, is mediated via inhibition in intestinal biotin uptake. This inhibition was associated with a significant reduction in expression of SMVT at the protein, mRNA, and hnRNA levels as well as a decrease in the activity of the SLC5A6 promoter in transgenic animals carrying this human construct. Chronic alcohol feeding also caused a significant inhibition in colonic uptake of biotin suggesting that uptake of the bacterially generated biotin is also negatively impacted by the chronic alcohol exposure. Similarly, findings were observed in studies utilizing human intestinal epithelial cells chronically exposed to alcohol. It is worthwhile mentioning that chronic alcohol exposure also inhibits biotin uptake by other cell types of the digestive system, namely, pancreatic acinar cells, as well as renal reabsorption process of the vitamin. The alcohol-mediated inhibition in biotin uptake in the case of pancreatic acinar cells was found to be mediated at the transcriptional level of the Slc5a6 gene and involves changes in the methylation status of the CPG islands predicted in the promoter of this gene and a decrease in the level of expression of the transcription factor KLF4,which plays an important role in regulating Slc5a6 transcription.
Other studies have shown that intestinal infection with Salmonella enterica serovar Typhimurium ( S. typhimurium ) inhibits gut biotin uptake, and that the inhibition is mediated via the action of proinflammatory cytokines. In the latter investigation, infecting mice with wild-type S. typhimurium (but not with its nonpathogenic isogenic invA spiB mutant) led to a significant inhibition in jejunal/colonic biotin uptake and in the level of expression of SMVT. No such inhibition in biotin uptake, however, was seen when cultured intestinal cells were infected with S. typhimurium suggesting that the effect of S. typhimurium infection in vivo is indirect and is likely mediated by proinflammatory cytokines; indeed, levels of these cytokines were found to be markedly induced in the intestine of the S. typhimurium -infected mice. Consistent with this suggestion was the observations that exposure of cultured colonic epithelial cells to the proinflammatory cytokines TNF-α and IFN-γ led to a significant inhibition in biotin uptake as well as in level of expression SMVT and activity of the SLC5A6 promoter. The inhibitory effect of proinflammatory cytokines on biotin uptake and on activity of the SLC5A6 promoter appeared to be mediated, at least in part, via the nuclear factor kappa-B signaling pathway.
Exposure of gut epithelia to the endotoxin lipopolysaccharide (a major component of the outer membrane of gram-negative bacteria that is released from bacterial cell walls by shedding or through bacterial lysis) has also been shown to lead to inhibition in colonic biotin uptake. This effect appears to be mediated via interference with the level of expression of the SMVT protein at the cell surface and is mediated via protein CK-2 (Lakhan R and Said HM; manuscript in preparation).
Other factors that interfere with the intestinal biotin uptake process are anticonvulsant drugs like carbamazepine and primidone.
54.8
Vitamin B 9 (Folate)
54.8.1
Metabolic Role and Effect of Deficiency
Folates refer to a group of one-carbon derivatives of the folic acid ( Fig. 54.1 ) that are required for the synthesis of pyrimidine and purine nucleotides, precursors of DNA and RNA, respectively. Folate is also involved in the metabolism of several amino acids including homocysteine. At the cellular level, folate deficiency leads to derangement of one-carbon metabolism, defect(s) in DNA synthesis and methylation, mis-incorporation of uracil into DNA, and disturbance in the metabolism of several amino acids. At the clinical level, folate deficiency and suboptimal levels are associated with a variety of abnormalities that include megaloblastic anemia, growth retardation, and neural tube defects in the developing embryo (the latter represents a relatively common birth defect in humans). In contrast, optimizing folate body homeostasis is associated with a marked reduction in the incidence of neural tube defects and may also provide protection against cardiovascular diseases and certain types of cancers. Folate deficiency/suboptimal levels are highly prevalent throughout the world and occur due to a variety of causes—including the impairment in intestinal absorption of the vitamin. A variety of conditions have been reported to affect/interfere with the normal intestinal folate absorption process such as the autosomal-recessive disorder “hereditary folate malabsorption syndrome” (HFMS), intestinal diseases (e.g., celiac disease, tropical sprue), drug interactions (e.g., sulfasalazine, trimethoprim, pyrimethamine, diphenylhydantoin), and chronic alcohol consumption.
54.8.2
Physiology of the Intestinal Folate Absorption Process
Two sources of folate are available to the gut: a dietary source (which is mainly processed and absorbed in the small intestine) and a gut microbiota-generated source. Dietary folates exist mainly in the free (i.e., folate monoglutamate) and polyglutamate forms, with the latter being the predominant form in the diet. The conjugated folate polyglutamates cannot be absorbed due to their size, multiple negative charges, and high hydrophilicity, and thus must be hydrolyzed to folate monoglutamate forms prior to uptake. This hydrolysis step is performed by the action of the enzyme folate hydrolase (also known as folylpoly-γ-glutamate carboxypeptidase). Two forms of the enzyme exist in intestinal epithelial cells. The first form is expressed at the apical BBM domain, and the second form is expressed intracellularly (most probably in the lysosomes). The BBM form of the enzyme is expressed mainly in the proximal part of the small intestine, while the intracellular form is expressed uniformly along the entire length of the small intestine. The intestinal BBM form of the folate hydrolase has been cloned and shown to be adaptively upregulated in folate deficiency ; this form is also developmentally regulated.
In describing the mechanism of folate uptake across biological membranes, it is important to mention here that the folate monoglutamate molecule is hydrophilic in nature and is negatively charged at physiological pH (p K a values for its α and γ carboxyl groups are 3.5 and 4.8, respectively). The mechanism of dietary folate monoglutamates uptake, by the small intestine (which also applies to the folate that enters the small intestine via the enterohepatic cycling of the vitamin ), has been extensively studied using a variety of human and animal intestinal preparations (reviewed in Ref. ). Collectively, these studies have shown that absorption of dietary folate monoglutamates occurs mainly in the proximal part of the small intestine and involves a specific and Na + -independent carrier-mediated mechanism. This mechanism, however, is highly dependent on acidic extracellular pH with a markedly higher uptake at acidic (pH 5–6) compared to neutral/alkaline (pH 7–7.4) pH. Studies with purified intestinal BBMV have also shown that the folate uptake system at the apical BBM domain is concentrative and is sensitive to the inhibitory effect of the anion transport inhibitors 4,4′-diisothiocyano-2,2′-disulfonic acid stilbene (DIDS), and acetamidoisothiocyanostilbene-2,2′-disulfonic acid. The effect of extracellular acidic pH on intestinal folate uptake is in part mediated via providing a trans-membrane pH gradient (suggesting the involvement of a folate − :H + cotransport mechanism and possibly a folate − :OH − exchange mechanism) and in part via a direct effect of the acidic extracellular pH on the activity of the carrier(s) involved. Other studies have shown that the intestinal folate uptake process has similar affinity for reduced (e.g., 5-methyltetrahydrofolate, 5-formyltetrahydrofolate), oxidized (e.g., folic acid), and substituted (e.g., methotrexate) folate derivatives. The mechanism of folate exit out of the intestinal absorptive cells, that is, transport across the BLM, has also been characterized using purified intestinal BLMV and again shown to involve a specific and DIDS sensitive carrier-mediated mechanism.
As to the microbiota-generated folate in the large intestine, previous studies have shown that a substantial portion of this folate exists in the absorbable monoglutamate forms and that the large intestine is indeed capable of absorbing some of this vitamin. The mechanism of folate absorption in the large intestine has been investigated using purified colonic AMV isolated from the colon of human organ donors and human-derived nontransformed colonic epithelial NCM460 cells. The results showed the involvement of an efficient and specific carrier-mediated mechanism. The identification of such an efficient uptake mechanism, for the microbiota-generated folate in the human large intestine, supports the belief that this source of folate may contribute to the overall nutritional requirement host for the vitamin,and especially to the cellular nutrition of the local colonocytes. Interferences with this mechanism may contribute to the development of localized folate deficiency and premalignant changes in the colonic mucosa.
54.8.3
Molecular Biology of the Intestinal Folate Uptake Process
Mammalian cells utilize three distinct and specific systems to transport folates across their plasma membrane. These systems are the ubiquitously expressed reduced folate carrier (RFC; the product of the SLC19A1 gene), which operates optimally at neutral/alkaline pH of 7.0–7.4 ; the transmembrane proton-coupled folate transporter (PCFT; the product of the SLC46A1 gene), which operates optimally at acidic pH 5.5–6.0 (with minimal activity at pH 7 and above) ; and the membrane anchored (via a glycosyl phosphatidyl-inositol linkage) folate receptors (FRs), which operates optimally at neutral pHs. While both the RFC and PCFT systems are expressed and functional in the intestinal tract, the FRs are not expressed in the normal intestine.
The human RFC (hRFC) polypeptide consists of 591 amino acids and is predicted to have 12 putative TMDs with both of the C- and N-terminals facing the cell interior. In addition, it has a large central intracellular loop that connects TM domains (TMDs) 1–6 and 7–12, three putative PKC phosphorylation sites, and one potential N-glycosylation site. The RFC protein is predicted to carry a net positive charge at physiological pH, which may be important for its interaction with the negatively charged substrate; also, the protein is N-glycosylated at position N58. The hRFC shares high degree of sequence homology with RFC of other mammals (e.g., mouse, rat, hamster, and chimpanzee). Functional identity of the cloned RFC has been verified by expression in different mammalian cells as well as Xenopus oocytes. RFC functions as an anion exchanger (apparent K m of 1–5 μM) moving the negatively charged folate molecule against its concentration gradient in exchange for downhill movement of an anion. Affinity of RFC is much higher for reduced folate derivatives (like the naturally occurring 5-methyltetrahydrofolate) than for oxidized folates (like the synthetic folic acid). The RFC protein is expressed at the BBM domain of the polarized enterocytes and at the apical membrane of colonocytes. Considering the optimal pH for function of this carrier and its distribution pattern along the intestinal tract, it is suggested that this transporter plays a role in folate uptake in the lower half of the small intestine and in the large intestine (where the pH at the intestinal surface is near the neutral range; Ref. ).
As to the hPCFT, this protein consists of 459 amino acids with 12 putative TMDs, two potential N-glycosylation sites, and with both the N- and C-terminals facing the cell interior. A critical role for PCFT in intestinal absorption of dietary folate has been established by the identification of loss-of-function mutations in the hPCFT gene in patients with HFMS, and in studies utilizing PCFT-knockout mouse model (Refs. and references therein). Folate transport mediated by PCFT is electrogenic and involves the net movement of a positive charge (proton), that is, it functions as a folate − :H + symport (Refs. and references therein). Folate is transported by this system against a concentration gradient utilizing the energy generated by the downhill movement of protons. The hPCFT mRNA is expressed mainly in the proximal part of the human small intestine with less expression in the distal small intestine and the large intestine. Furthermore, expression of the hPCFT is restricted to the apical membrane domain of the polarized enterocytes.
Since the PCFT and RFC systems are both expressed in the intestine (although at different levels in different regions of the gut) and both are localized at the apical membrane domain of the polarized intestinal epithelial cells, it is reasonable to conclude that both transporters contribute to overall gut folate absorption, but that the relative contribution is determined by the prevailing pH at the mucosal surface. Thus, PFCT is believed to play a predominant role in the absorption of dietary folate in the proximal half of the small intestine (where the pH at the luminal surface is around pH 5.8–6.0 as established by direct measurements with pH microelectrodes; 268), while RFC is believed to play a major role in folate absorption in the large intestine where the pH is in the neutral/slightly alkaline range.
With regard to the molecular identity of the folate transport system at the BLM domain of the intestinal absorptive epithelial cells, this is believed to be a member(s) of the multidrug-resistance-associated proteins (Ref. and references therein) although the apparent K m of these carriers is markedly higher than the levels of folate encountered by the intestinal BLM.
54.8.4
Cell Biology of the Intestinal Folate Uptake Process
Studies on the mechanisms involved in membrane targeting and intracellular trafficking of the hRFC and hPCFT proteins to the cell membrane have also been forthcoming. Using confocal imaging of live human intestinal epithelial cells and a series of truncated fusion proteins of hRFC with GFP (i.e., hRFC-GFP), the molecular determinants that dictate the targeting of the hRFC protein to the cell membrane were found to reside within the hydrophobic backbone of the polypeptide and not within its N- or C-terminal domains. Also, the integrity of the hRFC backbone was found to be critical for exporting the polypeptide from the endoplasmic reticulum to the cell surface. Numerous trafficking vesicles that contain the hRFC-EGFP fusion protein have also been identified and appear to be involved in the intracellular movement of the protein (real-time movies can be viewed at website: http://www.jbc.org.easyaccess2.lib.cuhk.edu.hk/cgi/content/full/277/36/33325/DC1 ). This intracellular trafficking process is dependent on the existence of an intact microtubule network; their disruption was shown to lead to a severe inhibition in the motility of the hRFC-containing trafficking vesicles as well as in the final expression of the hRFC protein at the cell membrane.
A recent study has identified a novel accessory protein that interacts with the hRFC in intestinal epithelial cells and influences its function ( Fig. 54.4 ). Using a bacterial two-hybrid system to screen a BacterioMatch II human intestinal cDNA library using the large intracellular loop between TMDs 6 and 7 of the hRFC as the bait, the dynein light-chain road block-1 (DYNLRB1) protein was identified as an interacting partner with hRFC. Existence of a direct protein-protein interaction between hRFC and DYNLRB1 was confirmed by in vitro pull-down assay, in vivo mammalian two-hybrid luciferase assay, and coimmunoprecipitation analysis. Moreover, confocal imaging of live human intestinal epithelial cells demonstrated colocalization of DYNLRB1 with hRFC. Coexpression of DYNLRB1 with hRFC led to a marked increase in folate uptake, while inhibiting the endogenous DYNLRB1 (with gene-specific siRNA or with vanadate, a specific pharmacological inhibitor) led to a marked decrease in the vitamin uptake.
With regard to cell biology of the hPCFT, live-cell confocal imaging studies have shown that the protein is expressed in a functional form at the apical membrane domain of epithelial cells and that its C-terminal sequence is not essential for membrane targeting or function. The latter investigations also showed that mutations that ablate a consensus beta-turn sequence separating predicted TM2 and TM3 abolishes apical folate uptake as a consequence of endoplasmic reticulum retention of mutant proteins. Furthermore, cell surface delivery of the hPCFT polypeptide was disrupted by microtubule depolymerization or by overexpression of the dynactin complex dynamitin (p50).
54.8.5
Structure-Activity Aspects of PCFT and RFC
Knowledge about structure – function activity of the hPCFT and hRFC systems has been emerging in recent years from clinical and experimental findings. Initial studies utilizing group-specific reagents have suggested possible involvement of positively charged histidine residues in intestinal uptake of the negatively charged folate. These histidine residues were believed to be located at or near the folate-binding site(s) of the involved folate uptake system(s). Subsequent molecule (site-directed mutagenesis) studies have confirmed a critical role for the conserved histidine residues located at positions 247 and 281 of the hPCFT polypeptide. In addition, clinical mutations identified in PCFT in patients with HFMS have implied a role for the amino acid residues located at positions 65, 66, 113, 147, 318, 376, and 425 of the polypeptide (Ref. and references therein). These mutations were shown to lead to spectrum of consequences that include an early stop codon and a frame shift (both of which lead to absence of hPCFT protein), or to a defective intracellular trafficking/membrane targeting of the protein and/or protein instability (Ref. and references therein). Other studies have reported a role for the residues located at positons 161, 232, 299, 304 in hPCFT function. Also, recent investigations have shown that the hPCFT forms homo-oligomers, which have functional significance, and that TMDs 2, 3, 4, and 6 play a role in the formation of these oligomers. Finally, experimental evidence was obtained to show that TMDs 1, 2, 7, and 11 forms an extracellular gate in PCFT in the inward-open confirmation.
With regard to RFC, this protein exists as homo-oligomer, but the monomer form appears to function independently (i.e., oligomerization is not a prerequisite for function but appears to be important for trafficking from the ER to the plasma membrane; Ref. and references therein). Many individual amino acid residues in the hRFC protein have been reported as being important for function including residues located at positions 29, 44, 45, 46, 48, 106, 107, 132, 133, 313, and 373 of the polypeptide (Ref. and references therein). Furthermore, studies have reported a functional role for the intracellular loop between TMDs 6 and 7, while no role was found for the N- or C-terminal tails of the RFC protein. Moreover, the membrane translocation pathway of the hRFC protein appears to be formed from TMDs 1, 2, 4, 5, 7, 8, 10, and 11 (reviewed in Ref. ).
54.8.6
Regulation of the Intestinal Folate Uptake Process
The intestinal folate uptake process is under the regulation of a variety of extracellular/environmental conditions and intracellular factors. Since a number of these conditions act via transcriptional mechanisms, it is important to first discuss what is known about the transcriptional regulation of the folate transport systems.
54.8.6.1
Transcriptional Regulation of the SCL19A1 and SLC46A1 Genes
Transcription of SLC19A1 (which encodes hRFC) appears to involve the activity of at least six alternative promoters that leads to the generation of 15 distinct 5′-untranslated regions (UTRs) but with a common hRFC open reading frame (reviewed in Refs. ). These promoters are regulated by both ubiquitous (SP, USF) and tissue-specific (e.g., AP1, C/EBP) nuclear factors leading to different levels of expression in different tissues and cell types. Evidence also exists to suggest that methylation and chromatin structure may affect the functionality of the hRFC promoters. The SLC19A1 promoter B appears to be responsible for driving the transcription of variant I, the predominant hRFC splice variant expressed in the human intestine.
With regard to the SLC46A1 gene, again the 5′-regulatory region (promoter) of this gene has been recently cloned and characterized. The minimal promoter of the SLC46A1 gene was mapped to a region that is 157 bp upstream of the ATG site and contains putative GC-box sites as well as enhancer elements (YY1 and AP1), which appear to play a role in promoter activity.
54.8.6.2
Adaptive Regulation of the Intestinal Folate Uptake Process
The intestinal folate uptake process is regulated by extracellular folate level. Dietary folate deficiency in rats leads to a specific and significant upregulation in transepithelial carrier-mediated folate uptake. This upregulation was mediated via an increase in the V max (with no changes in the apparent K m ) of the folate uptake process at the BBM and was associated with a parallel increase in expression of RFC and PCFT mRNA. Interestingly, the induction in the level of RFC mRNA was observed in the jejunum as well as in the ileum and colon. These findings suggest possible involvement of a transcriptional regulatory mechanism(s) in this adaptive upregulation in intestinal folate uptake process. The latter suggestion was subsequently confirmed for hRFC in studies using Caco-2 cells. In the latter studies, maintaining cells in a folate-deficient growth medium led to an upregulation in folate uptake, which was associated with an induction in hRFC protein and RNA levels. In addition, activity of the hRFC promoter B (the promoter that drives the expression of variant I of hRFC, that is, the predominant intestinal variant; Ref. ) was significantly higher in cells maintained in folate-deficient medium compared to those maintained in control growth medium. The folate deficiency-responsive sequence of the hRFC promoter B was found to be encoded in a sequence between − 2016 and − 1431 (i.e., outside the minimal promoter region required for basal activity which is encoded in a sequence between − 1088 and − 1043).
54.8.6.3
Developmental Regulation of the Intestinal Folate Transport Process
Transepithelial transport of folate in rat intestine undergoes clear changes during early stages of life. This was mediated via a progressive decrease in the V max (no changes in the apparent K m ) of the folate uptake process with maturation (i.e., from suckling to weanling to adult). These developmental changes were found in subsequent studies to involve the entry step of folate across the apical BBM of the polarized enterocytes. In addition, the changes were found to be associated with a parallel decrease in the level of the RFC protein and mRNA, as well as a decrease in the transcription rate of the SLC19A1 gene as indicated by results of Western blotting, RT-PCR, and nuclear run-on assays, respectively (Ref. ; level of PCFT was not determined in the latter study). These findings suggest that the developmental regulation of the intestinal folate uptake process occurs, at least in part, via a transcriptional regulatory mechanism(s). Additional studies are needed to determine the details of this mechanism and to determine the changes in level of expression of PCFT during early development.
54.8.6.4
Differentiation-Dependent Regulation of the Intestinal Folate Uptake Process
The intestinal folate uptake process also appears to undergo differentiation-dependent regulation as the cell moves from the crypt region to the villus tie. Studies using the human intestinal epithelial Caco-2 cells (which differentiate spontaneously in culture upon reaching confluence) showed a significant increase in carrier-mediated folate uptake with differentiation. This increase was associated with a marked increase in expression of hPCFT and hRFC at the protein, mRNA, and promoter levels. The latter findings suggest that this mode of regulation in intestinal folate uptake is (at least in part) mediated via transcriptional mechanism(s). Finally, the above-described findings with Caco-2 cells were confirmed in studies with native mouse intestine where folate uptake was found to be significantly higher in villus compared to crypt epithelial cells, which was again associated with a higher level of expression of PCFT and RFC at the protein and mRNA levels in the former compared to the latter cell type.
54.8.6.5
Regulation by Intracellular Signaling Pathways
Studies utilizing the human-derived colonic epithelial NCM460 cells and the rat-derived intestinal epithelial IEC-6 cells have reported the involvement of a PTK-mediated pathway in the regulation of intestinal folate uptake process. Treatment of these cells with specific inhibitors of the PTK-mediated pathway was found to lead to a significant inhibition in folate uptake. This inhibition appears to be mediated via a decrease in the activity (and/or number) of the functional folate carriers with no changes in their affinity. Similarly, an intracellular cAMP-mediated pathway appears to play a role in regulating intestinal folate uptake, but this regulation appears to be independent of the PKA-regulatory pathway.
54.8.7
Factors/Conditions That Negatively Impact the Intestinal Folate Uptake Process
It is well known that chronic alcoholism is associated with folate deficiency. While a variety of factors contribute to the development of this deficiency, an inhibition in intestinal absorption of folate is one of the significant contributors. Chronic alcohol use has been shown to affect both the initial hydrolysis phase of dietary folate polyglutamates and the subsequent absorption of the generated folate monoglutamates. The latter was associated with a significant reduction in folate uptake across the BBM and BLM domains of the absorptive epithelial cells as well as with a marked reduction in level of expression of RFC (level of PCFT was not investigated in these studies). It is interesting to mention here that a recent study examining the effect of chronic alcohol feeding on folate uptake by pancreatic acinar cells has shown significant reduction in the level of expression of both RFC and PCFT.
Other studies have reported an impairment in the activity of the intestinal folylpoly-γ-glutamate carboxypeptidase (folate hydrolase) in disease conditions that affect the intestinal mucosa (e.g., celiac disease and tropical sprue ), and as a result of chronic alcohol consumption and long-term use of the drug sulfasalazine.
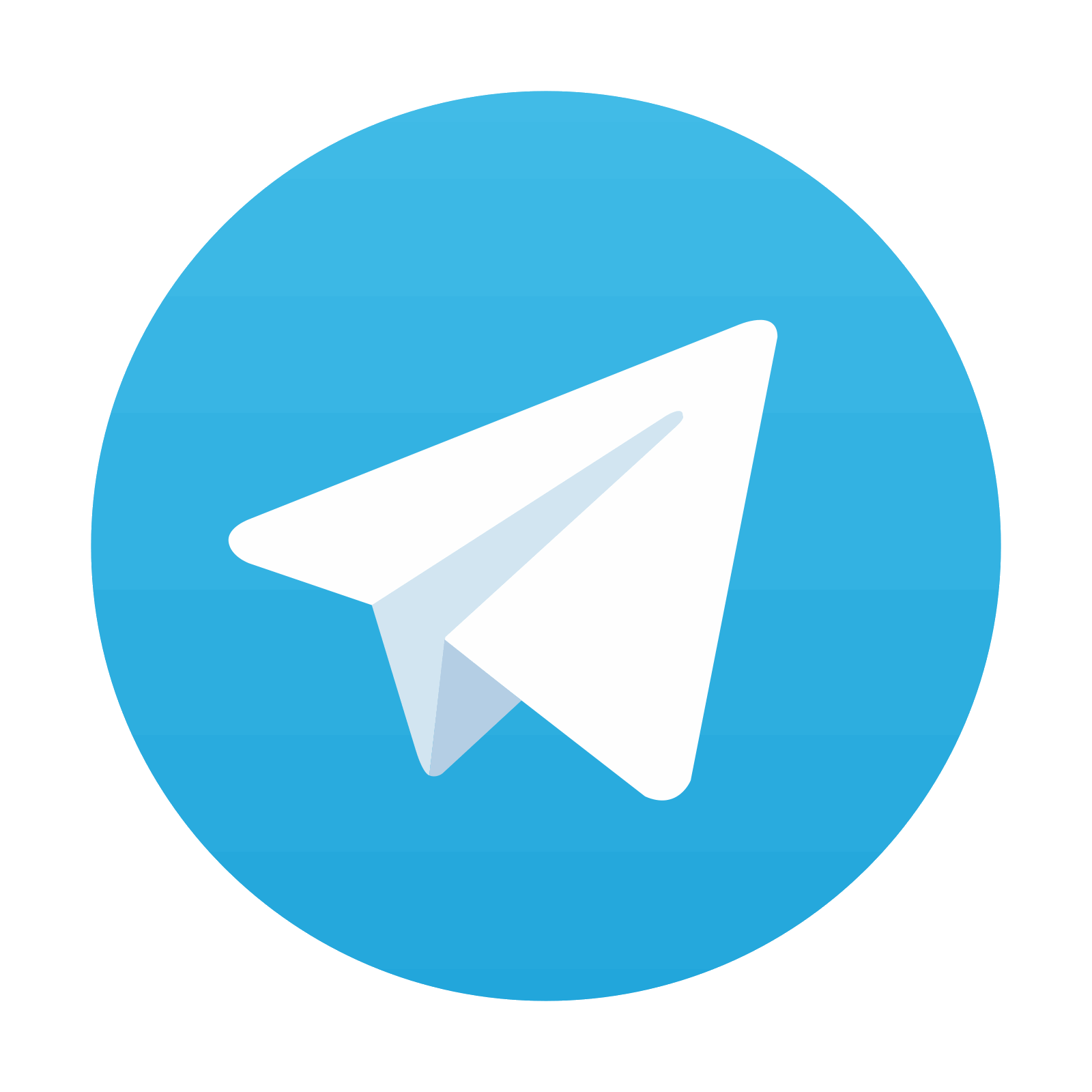
Stay updated, free articles. Join our Telegram channel
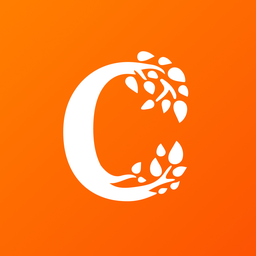
Full access? Get Clinical Tree
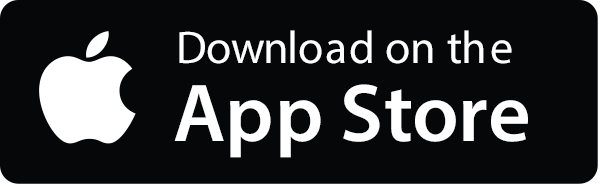
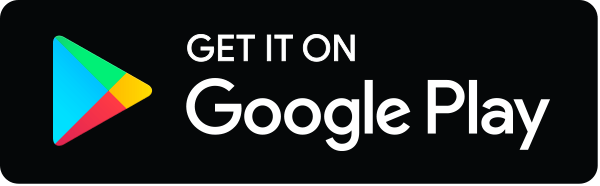