Researchers have estimated that the human body contains 10 14 cells, only 10% of which are not bacteria and belong to the human body proper. Furthermore, the number of genes in these microorganisms approximates 3.3 million, 150 times more than that of the human body. The mammalian intestinal tract represents a complex, dynamic, and diverse ecosystem of interacting aerobic and anaerobic, nonpathologic bacteria, and other microorganisms. This complex yet stable colony includes 400 to 500 distinct bacterial species.
Within any segment of the gut, some organisms are adherent to the epithelium, whereas others exist in suspension in the mucous layer overlying the epithelium. Binding to the epithelial surface is a highly specific process, and can be accomplished only by certain bacterial species. Bacterial adherence is also modulated by the local environment (i.e., pH), surface charge, and presence of fibronectin. Unbound bacteria within the lumen of the gut represent those organisms shed from the epithelium or swallowed from the oropharynx.
Luminal flora accounts for the majority of organisms within the gut; however, the fecal flora found in stool samples does not necessarily represent the important host–microbe symbiosis of the mucosal-bound flora. The majority of indigenous species are obligate anaerobes; therefore, their culture, identification, and quantification are technically difficult, and it is estimated that 99% of the indigenous bacteria cannot be cultured by using traditional methods. Limitations of conventional microbiologic techniques have confounded a detailed analysis of the enteric flora and led to a shift from traditional culture and phenotyping to genotyping. Modern techniques of ribotyping, pulsed-field electrophoresis, plasmid profiles, specific primers, and probes for polymerase chain reaction (PCR), nucleic acid hybridization, and 16S rRNA gene–dependent methods including terminal restriction fragment length polymorphism (TRFLP), fluorescent in situ hybridization, ribosomal intergenic spacer analysis, and DNA microarray have allowed for identification of bacteria without culturing. Such techniques are limited only by the number of probes developed to date to identify the bacteria of interest. Recent studies rely on the development of high-throughput sequencing of 16S rDNA hypervariable regions, which provide diversity and abundance of information on microbial communities.
Research efforts analyzing the symbiotic relationship that exists within the human gastrointestinal tract have been aided by studies of two well-described systems: the symbiosis between Rhizobium bacteria and leguminous plants, and the cooperative interaction between Vibrio fischeri and the light-producing organ of the squid. In each host tissue, modifications are made to allow a favorable niche to be established by the symbiont. The use of microbiologic techniques has helped to further elaborate the ways in which bacteria effect change within the host. For example, the use of laser capture microdissection and gene array analysis of germ-free mice colonized with Bacteroides thetaiotaomicron has shown effects on murine genes that influence mucosal barrier function, nutrient absorption, metabolism, angiogenesis, and the development of the enteric nervous system.
Host activities including processing of nutrients and regulation of the immune system are affected by the genetic potential of the indigenous flora, known as the microbiome. The composition of the intestinal microbiome is variable, and its diversity can be affected by alteration in diet and antibiotic use. Genes for specific metabolic pathways, such as amino acid and glycan metabolism, appear to be overrepresented in the microbiome of the distal gut, supporting the notion that human metabolism is an amalgamation of microbial and human processes.
Of the fungi, only yeasts play a major role in the orointestinal tract, with Candida being the predominant genus. Various strains are commonly, but not always, present in different locations, suggesting that they may be only transient flora. It has been found that up to 65% of individuals harbor fungi in the stool. The presence of Candida in the gastrointestinal tract does not indicate candidiasis. The colony counts of Candida in normal small and large bowel do not exceed 10 4 colony-forming units (CFU) per milliliter. The normal bacterial flora appears to provide protection against pathologic colonization by yeast. However, when the fecal flora is destroyed by antibiotics, then the yeast multiply. The addition of a Lactobacillus species to an in vitro system reduces the colony counts of Candida significantly. As opposed to the numerous indigenous bacterial flora and yeast forms, there does not appear to be a normal viral flora in humans.
Understanding the Indigenous Flora by Studying Germ-Free Animals
Further understanding of the beneficial effects of developing a normal bacterial flora is achieved by the analysis of germ-free animal models ( Box 4-1 ). Germ-free mice have small intestines that weigh less than those of their normal counterparts do. Their intestinal walls are thinner and less cellular; the villi are thinner and more pointed at the tip; and the crypts are shallower, resulting in a reduced mucosal surface area. Histologic evaluation shows that the mucosal cells are cuboidal rather than columnar and uniform in size and shape. The stroma has sparse concentrations of inflammatory cells under aseptic conditions, with only few lymphocytes and macrophages. Plasma cells are absent and Peyer’s patches are smaller with fewer germinal centers; consequently, there is little or no immunoglobulin A (IgA) expression. The T-cell component of the lamina propria is composed primarily of CD4+ lymphocytes; these are reduced in numbers in germ-free animals. Furthermore, antigen transport across the intestinal barrier is increased in the absence of intestinal microflora. Cellular turnover is decreased compared with colonized animals, and migration time for 3 H-thymidine-labeled mucosal cells from crypt to tip is doubled. After exposure to enteric bacteria, the intestines of germ-free animals take on a conventional appearance within 28 days, as one notes the infiltration of the lamina propria by lymphocytes, histiocytes, macrophages, and plasma cells.
Functional differences have also been noted in the intestines of germ-free animals including a more alkaline intraluminal pH and a more positive reduction potential ( E h ). Intestinal transit time and gastric emptying are also decreased in germ-free states. There is also increased absorption of calcium, magnesium, xylose, glucose, and some vitamins and minerals in the germ-free animal, as well as increases in the activity of intestinal cell enzymes, such as alkaline phosphatase, disaccharidases, and α-glucosidase.
Without a microflora, the rate of epithelial cell renewal is reduced in the small intestine, the cecum becomes enlarged, and the gut-associated lymphoid tissue (GALT) is altered. Studies have revealed that colonization of germ-free mice induces guanosine diphosphate (GDP)-fucose asialo-GM1 α1,2-fucosyltransferase activity in the epithelium; increased neutral glycolipid, fucosyl asialo-GM1; a decrease in asialo-GM1; and the production of Fuca1–2Gal structures. These changes occur selectively on the basis of specific bacterial strains and density. In studying the Rhizobium- legume symbiosis, researchers have learned that the soluble factors released by the bacteria signal a release of signaling molecules from the host, resulting in the expression of bacterial genes required for nodulation ( nod genes). These same genes have now been noted to be abnormal in Crohn’s disease and Blau syndrome.
A study evaluating the effect of the microbiome on mouse plasma biochemistry compared serum from germ-free and conventional mice. The study found a large number of chemical species in only the conventional mice. Amino acid metabolites were particularly affected. Multiple organic acids containing phenyl groups were also greatly increased in the presence of gut microbes. Specifically, at least 10% of all detectable endogenous circulating serum metabolites vary in concentration by at least 50% between the germ-free and conventional mice. Several of these molecules were either potentially harmful (e.g., uremic toxins) or beneficial (e.g., antioxidant).
Establishing the Indigenous Flora
Colonization of the newborn’s initially sterile gut with bacteria occurs within the first few days after birth. Such colonization appears to be rapid; indeed, bacteria have been found in meconium as early as 4 hours after birth. Initial inoculation in the majority of healthy infants are facultative anaerobes due to the positive oxidation–reduction potential of the neonates’ intestine. These bacteria including Staphylococcus spp., Enterobacteriaceae, and Streptococcus remain predominant during the first few days of life. The flora then rapidly changes and is affected by the mode of delivery (vaginal vs. cesarean section), maternal microbiota, week of gestation at birth, perinatal antimicrobial use diet (breast milk vs. formula), and perhaps maternal stress.
The study by Long and Swenson analyzed stool samples from 196 infants using traditional culture techniques and helped to define intestinal bacterial colonization with strict anaerobes, including Bacteroides fragilis . Among infants born vaginally, 96% were colonized with anaerobic bacteria within 4 to 6 days, with 61% harboring B. fragilis . In contrast, at 1 week in infants born full-term via cesarean section, anaerobes were present in only 59% and B. fragilis was found in 9%. The more recent KOALA Birth Cohort Study supported this finding by demonstrating that anaerobic colonization, especially Bacteroides spp., is delayed in cesarean section newborns, but that presence of Bifidobacterium spp. and Escherichia coli did not differ according to mode of delivery.
Colonization of the newborn can also be affected by maternal antibiotic use. A study by Gronlund et al. could find no permanent colonization with B. fragilis before 2 months of age among newborns born via cesarean with maternal prophylactic antibiotics. At 6 months of age, the colonization rate was 36%, half of that found in a group of vaginally born infants. Despite these differences in colonization, no differences in gastrointestinal signs such as flatulence, abdominal distension, diarrhea, foul-smelling stool, or bloody stools could be detected.
Infants born vaginally have been traditionally thought to acquire their fecal flora from the mother’s vaginal and intestinal flora. However, this has been called into question, with nosocomial/environmental spread appearing to be significant contributors. Within maternity wards, nosocomial spread of fecal bacteria among healthy newborns has been documented. Murono et al. studied the plasmid profiles of E. coli strains isolated from the stool of maternal and infant pairs to determine the degree of vertical versus nosocomial spread. In only 4 of the 29 pairs were shared Enterobacteriaceae documented. However, 8 of 10 infants in one hospital did share a single plasmid profile, indicating nosocomial acquisition of the fecal flora. Tannock et al. used the same plasmid profiling technique to show that Lactobacillus inhabiting the vaginas of mothers did not appear to colonize the infant digestive tract. Enterobacteriaceae and Bifidobacterium from the mother’s feces could be found to colonize the infant. The environment appears to play a greater role among infants born via cesarean section and those separated from their mother for long periods after birth.
As to the effect of age at birth on colonization, significantly fewer vaginally born preterm infants had anaerobes found in their stool at the end of 1 week, as compared with their vaginally born full-term counterparts, suggesting that either local conditions in the preterm infant’s intestine, such as lower acidity, or the sterile environment of an incubator affects colonization. Otherwise given the great interindividual variability among preterm infants with respect to factors such as maternal antibiotic treatment, diet, parenteral feeding, infection, and mechanical ventilation, there is little conclusive information about the development of their microbiota. Initial studies suggest that the flora of the preterm infant is of low diversity and contains a significant percentage of unknown species as well as potentially pathogenic species.
Breast-fed infants born vaginally had colonization similar to that of vaginally born formula-fed infants at 48 hours of age, indicating a similar “inoculum.” However, by 7 days, the flora of full-term breast-fed neonates is dominated by Bacteroides and bifidobacteria, which constitute 80% to 90% of the total microbial amount. This is in contrast to formula-fed infants, who had more complex flora with Bifidobacterium , enterobacteria, and Streptococcus in similar proportions. The formula-fed group also had greater numbers of Clostridium species. Of note, in infants whose diets were changed from a bovine formula to a soy formula because of allergy, there was no corresponding change in intestinal flora.
A study using bacterial enzyme activity as an indirect measure of bacterial colonization found no difference in flora during the first 6 months of life in infants who were delivered vaginally compared to infants delivered via cesarean section. However, stools collected from formula-fed infants had greater urease activity at 1 to 2 months and higher β-glucuronidase activity at 6 months compared with breast-fed infants. This is in contrast to the results of a study from Finland, in which no differences were found in enzyme activity according to feeding group. Examples of urease-producing fecal bacteria include Bifidobacterium , Clostridium , Eubacterium, and Fusobacterium . β-Glucuronidase producers include Lactobacillus, Clostridium, Peptostreptococcus , and E. coli . Multiple causes have been proposed for the differences in fecal flora observed between breast-fed and formula-fed infants, including the lower iron content and different composition of human milk (including various bifidogenic peptides, oligosaccharides, glycoproteins, and glycolipids ), a lower phosphate content, the large variety of oligosaccharides in human milk, and numerous humoral and cellular mediators of immunologic function in breast milk. Other factors include a lower colon pH in breast-fed infants, which promotes the growth of bifidobacteria and lactobacilli while inhibiting the growth of other bacteria. Finally, there may be a contribution from live bifidobacteria within human milk.
After infants are weaned, with the dramatic change in diet, the flora becomes more diverse and stable and resembles that of adults. A large European study by Fallani evaluated fecal samples of 605 infants 4 to 6 weeks after introduction of solids. At 4 weeks after introduction, bifidobacteria and Bacteroides still predominated. However, the numbers of Bifidobacterium , Clostridium perfringens, and Clostridium difficile decreased, whereas numbers of other strictly anaerobic clostridia increased. Of note even after 6 months, the influence of feeding method and mode of delivery persisted. By the age of 2.5 years, the microbiota fully resembles that of an adult in terms of composition. The period of maturation of the macrobiota may be critical for disease expression later in life.
Three different enterotypes have been described in the adult human microbiome, which are dominated by Prevotella, Ruminococcus , and Bacteroides . This microbiome remains stable until old age, when changes occur, perhaps due to digestive physiology and diet.
The role of diet in the composition of fecal flora in the older child and adult appears to be minimal, as individuals fed a standard institutional diet had similar fecal flora to those who consumed a random diet. The ingestion of an elemental diet resulted in reduction of stool weight and frequency, but few qualitative changes in the composition of the fecal flora. Furthermore, in analysis of the microorganisms measured in an aliquot of fresh feces, there does not appear to be significant differences in the fecal flora based on a diet’s fiber content or meat content. However, studies of the metabolic activity of the flora undertaken by evaluating bacterial enzymes have demonstrated marked differences in composition of colonic flora according to diet.
In contrast with earlier studies from the 1970s that showed colonization rates with E. coli in Western countries of at least 70% and in developing countries of nearly 100%, by the first week of life regardless of mode of delivery, a more recent Swedish study found E. coli colonization of less than 50%. The reduction was attributed to decreased nosocomial spread by the practice of “rooming-in” and early hospital discharge. It took almost 6 months before all infants were colonized with E. coli . The turnover rate of individual E. coli strains was low, most likely due to a limited circulation of fecal bacteria in the Swedish home. Environmental factors, such as siblings, pets, or feeding mode did not affect colonization kinetics.
Although some E. coli strains appear transient and disappear from the intestine within a few weeks, others become resident for months to years. Resident strains have certain characteristics such as the expression of P fimbriae, which allow bacteria to adhere to colonic epithelial cells. P fimbriae are composed of a fimbrial rod with a tip adhesion that exists in three papG classes (I, II, and III). These recognize the Galα1-4-Gal glycoproteins, with slight differences in binding. Intestinal persistence of E. coli has been linked to the class II variety of the adhesin. The resident strains more commonly have other virulence factors, such as the iron-chelating compound aerobactin and capsular types K1 and K5, when compared to the transient strains. Within the Swedish study, the P fimbrial class III adhesion gene associated with urinary tract infections was more common in E. coli from children who had cats in their home than in E. coli from children in homes without pets. This raises the question as to whether this E. coli could be transferred by close contact with a family cat.
Bacterial Flora within the Various Sections of the Gastrointestinal Tract
Oral Flora
Infants with a developing oral ecosystem are amenable to colonization perhaps because specific antibodies capable of inhibiting bacterial adherence are present only in low levels in early infancy. The indigenous microflora of the oral cavity is an integral component of the function of this site. The commensal bacteria help to defend against colonization by pathogens. Secretory immunoglobulin A (S-IgA) represents the main specific defense mechanism of the oral mucosa. The S-IgA of infant saliva and human milk are composed mainly of the IgA1 subclass. IgA proteases are produced by pathogenic bacteria as well as oral commensals. Saliva contains other immunoglobulins and defense factors to inhibit microbial adhesion and growth. After teeth emerge, IgG appears in greater concentrations. The early low concentrations of antibodies may be beneficial in allowing the invading bacteria to more easily colonize the oral surfaces. Initially only the buccal and palatal mucosa, as well as the crypts of the tongue, allow for colonization, but with the emergence of teeth, new gingival crevices and tooth surfaces become potential niches. Oxygen tension is an important environmental determinant for oral bacteria. The fastidious anaerobic growth even in edentulous mouths is explained by the formation of biofilms. Fusobacterium nucleatum , an obligate anaerobe, appears to play a crucial role in the maturation of oral biofilm communities.
The initial colonization of the oral cavity is dependent on mode of delivery, exposure to antibiotics, feeding mode, and age at birth. For example, the establishment of the primary bacterial group viridans streptococci is delayed in preterm infants and transiently compensated for by less prevalent inhabitants, such as yeast. The initial colonization by streptococci and Actinomyces allows for further colonization by other species. Initial bacteria are acquired through direct and indirect salivary contact during everyday activities; thus the colonies found within the oral cavities of young children often resemble those of the mothers. Viridans streptococci are the first persistent oral colonizers. The principal streptococcal species are Streptococcus mitis and Streptococcus salivarius . Oral actinomycetes (i.e., Actinomyces odontolyticus ) and various anaerobic species (i.e., Prevotella melaninogenica and F. nucleatum ) are also found during the first year of life. After the first year of life, the versatility among oral microflora increases remarkably. Among infants, there appears to be no stability among the specific clonal populations, and such instability is noted among adults, but to a lesser degree. This stability, or its lack, appears to vary according to the bacterial species being studied. Pathologic bacteria such as Streptococcus mutans , the main causative bacteria in dental caries, appear in the oral cavity only after the primary teeth emerge. Children colonized early by this bacterium are more susceptible to caries than those colonized later.
Esophageal Flora
Little is known about the bacterial colonization of the human esophagus. Because of the lack of anatomic or physiologic barriers to colonization, bacteria can be introduced into the esophagus either by swallowing oral flora or by reflux from a colonized stomach. Early attempts at defining the esophageal flora through samples obtained by luminal washing yielded poor results. Pei et al. used broad-range 16S rDNA PCR to examine biopsy samples from the esophagus of four healthy human adults. They identified 900 16S rDNA clones representing 41 genera of bacteria. Of these, 82.1% were cultivatable bacterial species, and there were about 10 4 bacteria per mm 2 mucosal surface of the distal esophagus. Members of six phyla—Firmicutes, Bacteroides, Actinobacteria, Proteobacteria, Fusobacteria, and TM7—were represented. The predominant bacterium was α-hemolytic Streptococcus species, and overall the flora was similar to that found in the oropharynx. A subsequent study by the same group defined a second microbiome with a greater proportion of gram-negative anaerobes/microphiles that correlated with disease states such as esophagitis and Barrett’s esophagus.
Stomach Flora
The stomach typically contains less than 10 3 CFU/mL, although these numbers appear to be higher in developing countries. The lower counts are attributed to gastric juices, which destroy most oral bacteria. Traditional culture methods identified gastric flora including Streptococcus, Neisseria, and Lactobacillus, as well as Candida . Newer biologic methods demonstrated that other microbes colonize the gastric mucosa, including Enterococcus, Pseudomonas, Staphylococcus , and Stomatococcus . Of interest, some of these studies washed the mucosal surface prior to sampling and found no change in the composition, suggesting these microbes are closely associated with the mucosa and not transient inhabitants.
Small Bowel Flora
The small intestine represents a transitional zone between the sparsely populated stomach and the exuberant bacterial flora of the colon. Accordingly, the proximal small bowel has bacterial counts similar to that of the stomach, with concentrations ranging between 10 3 and 10 4 CFU/mL in the duodenum and proximal jejunum. The predominant species are streptococci, staphylococci, and Lactobacillus . In addition, Veillonella and Actinomyces species are frequently isolated, but other anaerobic bacteria are present in lower concentrations. At the end of the transition, within the distal ileum, the gram-negative organisms outnumber gram-positive organisms. Here, anaerobic bacteria such as Bacteroides, Bifidobacterium, Fusobacterium , and Clostridium are found at substantial concentrations (e.g., 10 12 CFU/mL) along with coliforms. The distal ileum has an oxidation–reduction potential ( E h ) of −150 mV, which is similar to that of the cecum (−200 mV), thus allowing this portion of the small intestine to support the growth of anaerobic bacteria.
It is notable that small bowel concentrations are variable among animal species. Normal cats were noted to have relatively high numbers of bacteria (10 5 to 10 8 CFU/mL), including many obligate anaerobes in the proximal small intestine. This volume and composition was thought to be secondary to a strictly carnivorous diet.
Colonic Flora
Once in the colon, the bacterial concentrations increase dramatically. Colonic bacterial concentrations are typically 10 11 to 10 12 CFU/mL. Here anaerobic bacteria outnumber aerobes by 1000-fold. Predominant species include Bacteroides, Bifidobacterium , and Eubacterium , with anaerobic gram-positive cocci, Clostridium , enterococci, and various Enterobacteriaceae also common.
Controlling the Growth of the Indigenous Population
Various host defenses are responsible for controlling the proliferation of intestinal bacteria, thereby limiting the population size ( Box 4-2 ). Such limitation is needed because under optimal conditions in vitro coliform bacteria can divide every 20 minutes. If this were to occur in vivo the host would quickly become overwhelmed. Within the gastrointestinal tract, bacterial generation time is longer, at one to four divisions per day. Within the small intestine, the major defenses against bacterial overgrowth are gastric acid and peristalsis. The ability of the peristaltic wave to propel bacteria is inferred by Dixon’s classic study in which a surgically created subcutaneous loop of rat small intestine was inoculated by 51 Cr-labeled red blood cells (RBCs) and bacteria. The bacteria and RBCs were noted to be rapidly cleared from the small intestine by the rat’s peristaltic activity. The effectiveness of peristalsis in moving bacteria is further emphasized by those circumstances in which bacterial overgrowth is found in a loop of intestine with ineffective peristalsis. In addition, gastric acid has been shown to contribute to the sparse bacterial colonization of the proximal intestine. Gram-negative organisms are particularly susceptible to the effects of a low pH, and a large inoculum of Serratia organisms is eradicated within 1 hour when in contact with normal gastric acidity. Indeed, patients with achlorhydria harbor coliforms and anaerobic gram-negative bacilli in the proximal small bowel, as well as increased numbers of streptococci, Lactobacillus , and fungi. Lowering of gastric acid pharmacologically has been shown to impair host defenses against pathologic bacteria including Vibrio cholerae , Candida , Campylocacter , and Strongyloides stercoralis .
Host Factors
Intestinal motility/peristalsis
Gastric acid
Antibacterial quality of pancreatic and biliary secretions
Intestinal immunity (IgA, Paneth cell products [defensins], lysozyme, bactericidal permeability increasing protein), epithelial cell products
Mucous layer
Microbial Factors
Alteration in redox potential
Substrate depletion
Growth inhibitors (short-chain fatty acids [SCFAs], bacteriocins)
Suppression of bacterial adherence
Bile duct ligation in experimental animals results in cecal overgrowth with coliforms, suggesting that bile acids or some other component of bile plays a role in the regulation of the bacterial flora. It is suspected that the deconjugation of bile acids by the indigenous flora to create simple bile acids with the ability to inhibit bacterial growth is a possible mechanism.
Microbial interactions constitute a major factor in regulating the indigenous microflora, particularly within the colon. Various interactions can either promote or inhibit growth of organisms. One mechanism would be competition for substrates. An example is the inhibition of the growth of Shigella flexneri by coliform organisms that compete for carbon. Another mechanism would be manipulation of the oxygen content of the environment. The maintenance of a reduced environment by facultative bacteria allows the growth of anaerobic bacteria. By-products of bacterial metabolism can create an intraluminal environment that restricts growth. Short-chain fatty acids (SCFAs)s such as acetic, propionic, and butyric acid can inhibit bacterial proliferation. At sufficiently low pH, these acids are undissociated and can enter the bacterial cell to inhibit microbial metabolism. Lactobacillus spp., particularly Lactobacillus plantarum , are found throughout the gastrointestinal tract, and their ability to adhere to mannose-containing receptors on epithelial cells is important in protecting against colonization by pathogens. Finally, some bacteria can produce antibiotic-like substances termed bacteriocins, enocin, and hydrogen peroxide, which can inhibit the growth of other bacterial species or even contribute to self-regulation.
Mucus is secreted by the colon (primarily mucin 2 [MUC2]) and forms a loose outer layer that contains bacteria, and an inner dense layer that is sterile. The outer layer is propagated along the intestinal tract, whereas the inner layer forms a physical and chemical barrier between the microbial population and the epithelial cells. This inner layer contains antimicrobial peptides, enzymes, and enteric organism-specific secretory IgA, IgM, and IgG. The carbohydrate component of mucin can compete for receptor–specific binding proteins of microbes.
Host immunity also plays a role in limiting the growth of the indigenous bacterial population. IgA synthesis by B cells of GALT is stimulated by the endogenous flora and increased further with pathologic colonization as in Shigella infection or bacterial overgrowth. Distinct B-cell populations secrete different types of IgA, which may help control the volume and composition of the flora. Such IgA is thought to prevent bacterial adhesion to epithelial cells. However, isolated IgA deficiency is not associated with alterations in the pattern of colonization. Moreover, the acquisition and composition of T- or B-cell–deficient mice is indistinguishable from that of their immunologically intact littermates. Paneth cells of the small intestine secrete antibacterial peptides called defensins that have antibacterial properties, as well as phospholipase A2, bactericidal permeability-increasing protein, and lysozyme.
The pattern of antibodies directed against fecal bacteria appears to be unique for each individual. People tend to make antibodies against both indigenous bacteria and transient bacteria. The antibodies include polyspecific IgM as well as specific IgG and IgA. Relatively more-specific IgA antibodies appear to be directed against transient bacteria in comparison to indigenous bacteria.
Symbiosis Between Host and Fecal Flora
A microflora-associated characteristic (MAC) is defined as the recording of any anatomic structure or physiologic or biochemical function in a host organism that has been influenced by the microflora. When such changes occur in the absence of microflora, they are designated as a germ-free animal characteristic (GAC). The distinction between MAC and GAC helps to define the symbiotic relationship that exists between human and the microbial host, and elucidates those processes that bacteria perform that are advantageous to the host ( Box 4-3 ). Bacterial β-glucuronidase and sulfatase are responsible for the enterohepatic circulation of numerous substances including bilirubin, bile acids, estrogens, cholesterol, digoxin, rifampin, morphine, colchicine, and diethylstilbestrol. Microflora also plays a role in the degradation of intestinal mucin, the conversion of urobilin to urobilinogen, and of cholesterol to coprostanol, and the production of SCFAs. Mucin-degrading microbes are evident in all children by age 20 to 21 months. This appears to be a gradual acquisition process starting at about 3 months of age. Bacterial synthesis of vitamins such as biotin, vitamins K and B 12 , pantothenate, riboflavin, and folate help supplement dietary sources. Bacterial enzymatic degradation of urea is probably the only source of ammonia in the animal host.
Beneficial Effects
Competitive exclusion of pathogens
Production of short-chain fatty acids
Synthesis of vitamins and nutrients
Enterohepatic circulation of numerous substances (e.g., bilirubin, bile acids, estrogens, cholesterol, digoxin, rifampin, morphine, colchicine, and diethylstilbestrol)
Degradation of intestinal mucin
Conversion of urobilin to urobilinogen
Conversion of cholesterol to coprostanol
Degradation of urea
Drug metabolism and activation
Development of the immune system
Development of the enteric nervous system
Detrimental Effects
Competition for calories and essential nutrients
Production of harmful metabolites (carcinogens, deconjugated bile acids, hydroxyl fatty acids)
Mucosal damage
Direct effect of bacteria
Exacerbate inflammatory disease
A broad spectrum of metabolic reactions have been performed by intestinal flora, including hydrolysis, dehydroxylation, decarboxylation, dealkylation, dehalogenation, deamination, heterocyclic ring fission, reduction, aromatization, nitrosamine formation, acetylation, esterification, isomerization, and oxidation. Gut flora acts on drugs to result in activation, toxin production, or deactivation. The bioavailability and pharmacologic effect of numerous drugs, such as opiates, digoxin, hormones, and antibiotics, have been demonstrated to be altered by gut flora. β-Lyases transform xenobiotic cysteine conjugates to toxic metabolites such as thiols or thiol derivatives. The azoreductase activity of the colonic flora metabolizes the prodrug sulfasalazine to its active aminosalicylate.
SCFA production is thought to occur in the cecum and ascending colon, mainly by the anaerobic flora. It appears that those infants fed breast milk produce fewer SCFAs than those fed formula, in which there is a more varied, adult-like SCFA profile. SCFAs produced in the colon may represent up to 70% of the energy available from the ingestion of carbohydrate.
Intestinal microflora enzymes β-glucuronidase and sulfatase catalyze the deconjugation of estrogens excreted with bile into the intestine to allow for reabsorption as part of the enterohepatic circulation. The suppression of the intestinal microflora with antibiotics results in a decrease in the enterohepatic circulation of sex steroids and can thus lower the concentrations of these hormones significantly. Failed oral contraception has been linked to concomitant use of antibiotics.
Bile acids are derived from cholesterol in the liver. Within the liver, primary bile acids are conjugated and excreted into the bile. Bile acids undergo enterohepatic circulation several times each day. Most of the absorption takes place by active transport in the terminal ileum. In the intestine, conjugated bile acids are acted on by bacterial enzymes and converted to secondary bile acids. These secondary bile acids are either excreted into the feces or absorbed and sometimes further metabolized within the liver into tertiary bile acids. Microbial transformation of bile acids includes deconjugation, desulfation, deglucuronidation, oxidation of hydroxyl groups, and reduction of oxo groups. Because humans are born germ free, primary bile acids can be found in the meconium of newborn babies. Short-chain bile acid levels are elevated in children and adults with cholestasis. In healthy children, the levels of short-chain bile acids are undetectable. The ability to hydrolyze taurine and glycine bile acid conjugates has been detected in Bifidobacterium , Peptostreptococcus , Lactobacillus , and Clostridium in infants shortly after birth. The occurrence, substrate specificity, and kinetics of this enzyme activity vary among species and bacterial strains. Jonsson et al. observed a decrease in sulfated conjugates within the stool at approximately 6 months of age. Because this occurred at the same time that sulfate-rich mucin disappeared, they suspected that this decrease was caused by the action of microbial desulfanates. Two clostridial strains ( Clostridium spp. S1 and S2) and Peptostreptococcus niger H4 desulfate bile acid 3-sulfates. Jonsson et al. also noted that by 24 months of age, all the children studied had an adult pattern of excreted bile acids, in that they were lacking a hydroxyl group at C-7. Bacteria that are known to have 7α-dehydroxylation activity include Eubacterium , Clostridium , and Lactobacillus . Cholesterol elimination is accomplished by two major routes: conversion of cholesterol to coprostanol and 7α-dehydroxylation of bile acids. Infants appear to be unable to perform such elimination during the first several months of life. Thus, during those months, sulfation appears to be a compensatory mechanism for the excretion of breakdown products of cholesterol.
Certain commensal bacteria are known to produce neurotransmitters and neuromodulators, which can affect gut sensation and motility. Indeed, there is a suggestion that the microbiota can influence the development and function of the central nervous system leading to the concept of the microbiota-gut-brain axis.
Bacterial Flora in Illness
Pathologic colonization occurs with the same species that predominate in nosocomial infections, and studies suggest that colonization is a risk factor for infection. This is the theory behind prophylactic decontamination of the digestive tract in the critically ill, which has been shown to reduce mortality. Changes in the composition of the gut flora are common in critical illness due to reduced enteral intake, reduced intestinal motility, use of acid blockade therapy, and use of broad-spectrum antibiotics. Gram-negative organisms are rarely found in the oropharynx of healthy individuals, yet they can be found in up to 75% of hospitalized patients. Similarly, du Moulin et al. documented the effects of antacids on the flora of the stomach. Among 59 critically ill patients, simultaneous colonization of the gastric and respiratory tract was seen with aerobic gram-negative bacteria. This and similar studies have been the basis of the controversy surrounding routine acid blockade therapy for critically ill patients. Overall, it appears as though only in selected patients does the benefit of stress ulcer prophylaxis outweigh the risk of nosocomial pneumonia. Gastric colonization in these patients also appears to be a risk factor for wound infections, urinary tract infections, peritonitis, and bacteremia. Results have been varied of studies aimed at decreasing bacterial overgrowth via selective decontamination of the digestive tract using topical, nonabsorbed antimicrobial agents active against aerobic gram negatives (tobramycin and polymyxin) and fungi (amphotericin), but leaving gram-positive flora to preserve colonization resistance. However, a meta-analysis indicates that this strategy is effective in preventing nosocomial respiratory infection and reduces intensive care unit (ICU) mortality.
Total parenteral nutrition given to experimental animals increased the concentration of aerobic gram-negative organisms in the cecum and bacterial translocation into lymph nodes when compared with enterally fed animals. Indeed, enteral feeding in the critically ill human is associated with fewer nosocomial infections.
Bacterial Flora and Allergy
Although the exact pathophysiology of allergic disease is incompletely understood, it is thought to represent the result of disordered function of the immune system. The intestinal barrier in infants is thought to be immature and thus vulnerable to allergic sensitization during the first few months of life. The intestinal microflora strengthens the immune defense and stimulates the development of the gut immune system. In newborns, the type 2 T helper cell (Th2) cytokines, essential mediators in the formation of allergic inflammation, predominate over Th1 cytokines. Th2 cytokines include interleukin 4 (IL-4), which induces B-cell differentiation into IgE-producing cells, and IL-5, which is important for eosinophil activity. Intestinal bacteria can counterbalance this Th2 activity and promote the development of the Th1 cell lineage, and thus regulate the IgE response. This may be the result of the CpG motif, which can induce polyclonal B-cell activation and secretion of Th1 cytokines such as IL-6, IL-12, and interferon (IFN). Intestinal bacteria may also modulate allergic inflammation via modification of antigen uptake, presentation, and degradation. Thus, in those children with an aberrant array or insufficient number of intestinal microorganisms, there may be an inability to strengthen the gut barrier or counterbalance a Th2 cytokine profile. This inability to reduce the two major risk factors toward developing allergy may lead to sensitization. The role of bacteria in the formation of allergy is strengthened by clinical studies demonstrating that there are differences in the microflora between allergic and nonallergic individuals. One study revealed that nonallergic individuals had higher counts of aerobic bacteria during the first week of life, as well as greater numbers of Lactobacillus at 1 month and 1 year of age. At age 1 to 2 years, the allergic children have greater prevalence of Staphylococcus aureus and Enterobacteriaceae and fewer Bacteroides and Bifidobacterium . Allergic children also appear to have greater number of Clostridium at 3 weeks of age. In another study, allergic infants were found to have high levels of the adult-type bifidobacteria ( Bifidobacterium adolescentis ) compared with healthy infants who had greater numbers of Bifidobacterium bifidum . A comparison of the adhesive properties of these two strains found that the adhesive abilities of B. bifidum were significantly greater. These results suggest that the greater adhesive qualities may help to stabilize the mucosal barrier and prevent absorption of antigenic proteins. In the Copenhagen Prospective Study on Asthma and Childhood, which followed a birth cohort of 411 high-risk children for 6 years, researchers found that reduced bacterial diversity of the infant’s intestinal flora was associated with increased risk of allergic sensitization, increased peripheral blood eosinophil counts, and allergic rhinitis, but not asthma or atopic dermatitis.
Lifestyles that limit antibiotic use and encourage the ingestion of fermented foods appear to result in a decreased risk of developing allergy. Similarly, the early use of antibiotics appears to be a risk factor for developing later atopic disease, although results of a large Dutch cohort study suggest that such early antibiotic exposure may predispose an individual to wheezing but not to the development of eczema. Inflammation is triggered by Toll-like receptors (TLRs), a group of evolutionarily conserved pattern-recognition receptors that are present in intestinal epithelial cells and antigen-presenting cells. More than 10 members of the TLR family have been a described, each of them possessing specificity toward microbial surface-structure elements.
Bacterial Flora and Antibiotics
Nearly all antibiotics have an effect on the bacterial flora. The effect is dependent on the intraluminal concentration, as well as the antimicrobial spectrum. Such an effect can be advantageous, and numerous studies have demonstrated the reduction of wound infections following surgery with the use of prophylactic antibiotics. Among neutropenic patients, intestinal colonization with gram-negative aerobic bacilli, especially Pseudomonas aeruginosa , frequently precedes infection. Use of prophylactic antibiotics to modify the intestinal flora has been shown to reduce the incidence of infection in this population.
The use of oral ampicillin or penicillin suppresses the normal aerobic and anaerobic flora including Bifidobacterium , Streptococcus , and Lactobacillus spp., and causes overgrowth of Klebsiella , Proteus , and Candida spp. However, administration of cefaclor, an oral cephalosporin, and cephalexin appears to cause little change, except for a reduction in Enterobacteriaceae. Erythromycin administration results in fewer marked changes than observed with penicillins; however, there is a significant decrease in Enterobacteriaceae. Oral gentamicin administration results in drastic changes including a marked decline in E. coli . However, intravenous gentamicin is excreted into the intestine with bile at lower concentrations and thus alters the flora only slightly. Cefpiramide, a parenteral expanded-spectrum cephalosporin, which is excreted in the bile at high concentrations, suppresses normal flora so markedly that almost all species of organisms are eradicated and the active growth of yeast is promoted. There appears to be a rapid return of the disturbed flora to normal levels within 3 to 6 days after therapy, although a minority of researchers believe recovery time could be longer, on the order of 2 weeks or more. Suppression of the normal flora results in lowered colonization resistance and promotes overgrowth of resistant organisms, in addition to allowing for colonization with pathogens such as C. difficile .
Antibiotics may also affect fecal bulk. Volunteers on a constant diet who were administered ampicillin and metronidazole were noted to have a 97% increase in their fecal bulk. This was accompanied by a 69% increase in fecal fiber. The author suggests that the absence of digestion of the fecal fiber by the indigenous flora was the mechanism by which the antibiotics resulted in increased fecal bulk.
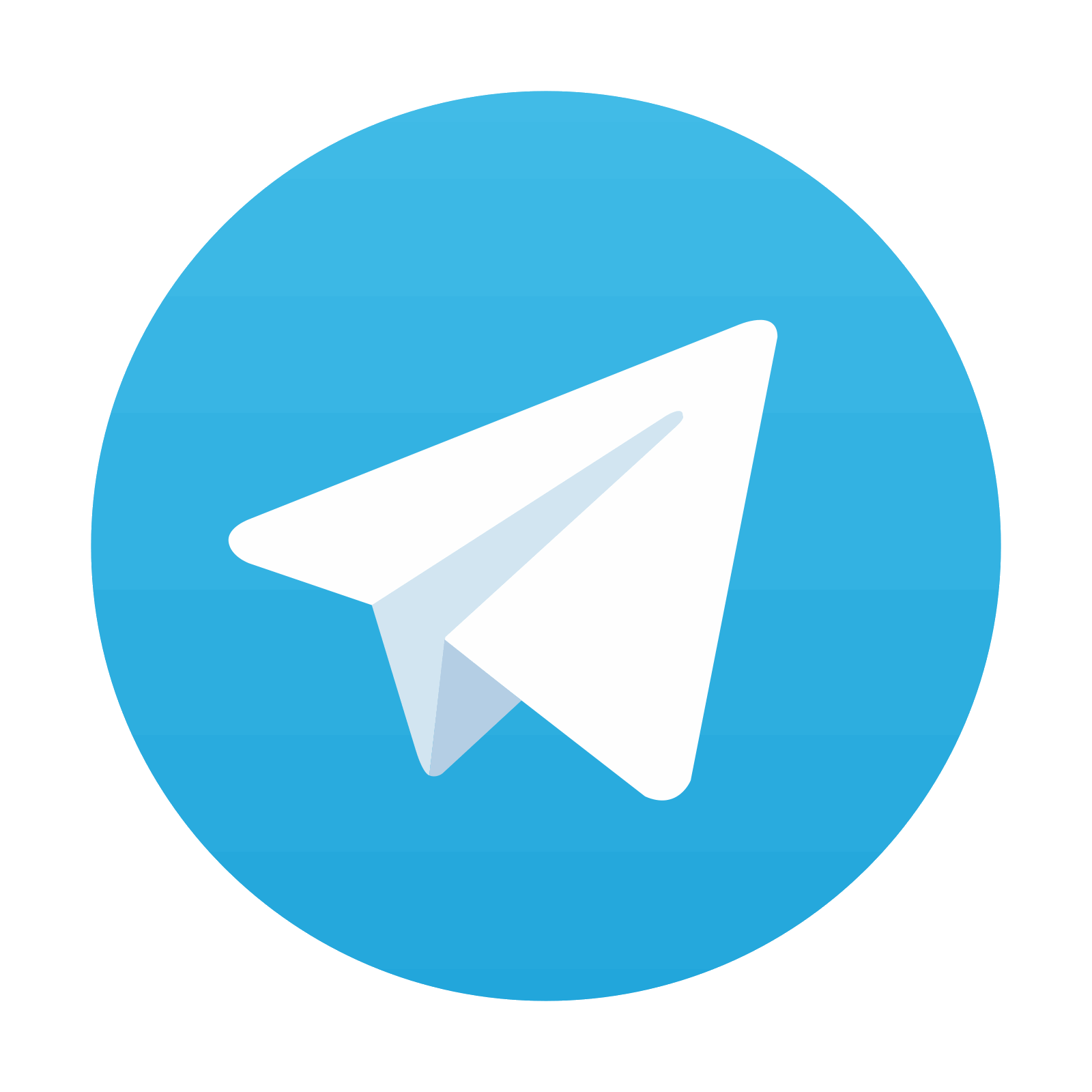
Stay updated, free articles. Join our Telegram channel
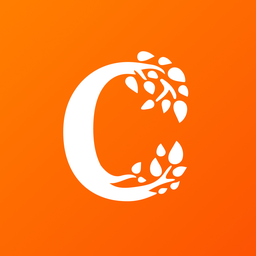
Full access? Get Clinical Tree
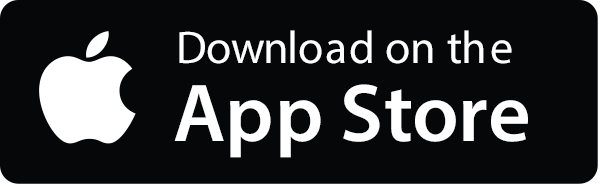
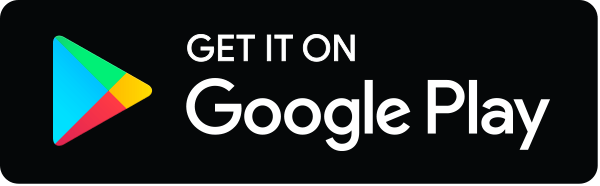