Despite advances in therapy, hepatitis C virus infection remains a major global health issue with 3 to 4 million incident cases and 170 million prevalent chronic infections. Complex, partially understood, host-virus interactions determine whether an acute infection with hepatitis C resolves, as occurs in approximately 30% of cases, or generates a persistent hepatic infection, as occurs in the remainder. Once chronic infection is established, the velocity of hepatocyte injury and resultant fibrosis is significantly modulated by immunologic as well as environmental factors. Immunomodulation has been the backbone of antiviral therapy despite poor understanding of its mechanism of action.
Key points
- •
Disruption of the generation of type I and III interferons (IFN), such as IFN-α, IFN-β, and IFN-λ, is critical for establishment of persistent infection of hepatocytes. As such, the specific viral particles specifically inhibit several pathways required for generation of and response to secreted interferons.
- •
The efficacy of dendritic cell antigen presentation to adaptive B- and T-cell effectors determines whether brisk and effective response resolves acute infection or delayed and weak responses allow chronic infection.
- •
Multiple nonparenchymal cells in the liver can either contribute to antiviral effects or induce tolerance by T cells.
- •
Regulatory T cells play a fundamental role in suppressing early antiviral responses by T and B cells but may play a critical protective role against rapid liver injury in chronic infection.
- •
Neutralizing antibodies produced by B cells do not universally resolve early infection nor protect against reinfection and can even facilitate viral entry into hepatocytes.
Introduction
Despite advances in therapy, hepatitis C virus (HCV) infection remains a major global health issue with 3 to 4 million incident cases and 170 million prevalent chronic infections. Complex, partially understood, host-virus interactions determine whether an acute infection with hepatitis C resolves, as occurs in approximately 30% of cases, or generates a persistent hepatic infection, as occurs in the remainder. Once chronic infection is established, the velocity of hepatocyte injury and resultant fibrosis is significantly modulated by immunologic as well as environmental factors. Although the backbone of antiviral therapy for most of the past 15 years has consisted of innate immune stimulation with interferon-α (IFN-α), more recent data regarding of the role of T-cell exhaustion and antigen-presenting cell defects have encouraged early-phase clinical trials of several novel immunomodulators with modest initial effects. An appreciation of the complexity, redundancy, and interdependence of the regulatory mechanisms involved in maintaining chronic infection may inform future approaches to restore immunoreactivity to HCV as feasible antiviral therapy. This review focuses on virus-induced immunologic dysfunction that allows the establishment of persistent infection as well as the impact of these immunologic defects on disease progression during chronic infection.
Introduction
Despite advances in therapy, hepatitis C virus (HCV) infection remains a major global health issue with 3 to 4 million incident cases and 170 million prevalent chronic infections. Complex, partially understood, host-virus interactions determine whether an acute infection with hepatitis C resolves, as occurs in approximately 30% of cases, or generates a persistent hepatic infection, as occurs in the remainder. Once chronic infection is established, the velocity of hepatocyte injury and resultant fibrosis is significantly modulated by immunologic as well as environmental factors. Although the backbone of antiviral therapy for most of the past 15 years has consisted of innate immune stimulation with interferon-α (IFN-α), more recent data regarding of the role of T-cell exhaustion and antigen-presenting cell defects have encouraged early-phase clinical trials of several novel immunomodulators with modest initial effects. An appreciation of the complexity, redundancy, and interdependence of the regulatory mechanisms involved in maintaining chronic infection may inform future approaches to restore immunoreactivity to HCV as feasible antiviral therapy. This review focuses on virus-induced immunologic dysfunction that allows the establishment of persistent infection as well as the impact of these immunologic defects on disease progression during chronic infection.
Intrahepatocytic sensing and signaling
HCV is a highly mutable, hepatotropic, enveloped single-stranded RNA virus of the Flaviviridae family. The 9.5-kB genome encodes for a single polyprotein of 3011 amino acids flanked by 5′ and 3′ noncoding regions essential for viral replication. The viral polyprotein is translated in toto and then processed by host cell- and virus-encoded proteases into structural (Core, E1, E2) and nonstructural proteins (p7, NS2, NS3, NS4A, NS4B, NS5A, NS5B). The Core protein forms the viral nucleocapsid. E1 and E2 are the viral envelope glycoproteins that interact with various surface receptors, including claudin-1, CD81, DC-SIGN, scavenger receptor B I (SR-BI), and the LDL receptor, to mediate viral entry into target cells. The remainder of the nonstructural proteins creates a membrane-associated viral replication complex critical for establishment for persistent, and along with structural proteins, modulates host cell function to disrupt intracellular and extracellular antiviral pathways ( Table 1 ).
Core | Activation of PKR |
Inhibition of TLR3-mediated secretion of type I and III interferon | |
Inhibition of STAT1 activation | |
Inhibition of ISGFR3 nuclear translocation | |
Impairment of dendritic cell maturation and antigen presentation | |
Blockade of IL-1β production in macrophages | |
Stabilization of HLA-E | |
C1q-mediated T-cell inhibition | |
E2 | Activation of PKR and elongation initiation factor 2a (eIF2a) |
Stimulation of CD81 on B cells | |
NS2 | Inhibition of TBK1 and IKKe |
NS3/4A | Cleavage of MAVS |
Inhibition of TBK1 and IKKe | |
Cleavage of TRIF | |
Suppression of CXCL8 and CXCL10 chemokines | |
NS5A | Activation of PKR |
NS5B | Viral mutations leading to escape or inhibition of T-cell function |
The induction of type I (IFN-α and IFN-β), II (IFN-γ), and III (interleukin [IL]-28A/IFN-λ2, IL-28B/IFN-λ3, IL-29/IFN-λ1, and IFN-λ4) interferons is critical for host-defense against intracellular pathogens. Type I, II, and III IFNs signals in autocrine and paracrine manners through their respective heterodimeric receptors (IFNAR, IFNGR, IFNLR) to phosphorylate STAT-1 and STAT-2, leading to the formation of the transcription factor ISGF3 (consisting of pSTAT-1, pSTAT-2, and Interferon Response Factor [IRF] 9) that translocates to the nucleus to modulate the production of hundreds of IFN-stimulated antiviral genes (ISGs). Specific ISGs suppress viral replication and sensitize infected cells to apoptosis. Critical to the development of adaptive immune responses, type I interferons also stimulate immunoproteasome formation critical for presentation of antigen by hepatocytes to CD8 T cells. Although IFNAR and IFNGR expression is ubiquitous, the heterodimeric IFNLR (consisting of IFN-λR1 and IL-10R2 chains) is restricted to epithelial cells, hepatocytes, and dendritic cells (DCs). Although there is significant overlap in the number and types of genes induced by type I, II, and III interferons, IFN-α and IFN-λ do have subtle differences in gene expression profiles and kinetics. Notably, the permissiveness of hepatocyte cell lines to support HCV infection in vitro appears critically dependent on defects in type I and III interferon signaling.
After viral entry and uncoating, hepatocytes sense intracellular HCV infection to generate type I and III interferons both by toll-like receptors (TLRs) and by retinoic-acid-inducible gene-like receptors, a family of proteins that includes retinoic acid-inducible gene I (RIG-I), melanoma differentiation-associated gene 5 (MDA-5), and laboratory of genetics and physiology gene 2 (Lgp2), in the cytoplasm. TLR3 recognizes double-stranded RNA replication intermediates on the cell membrane or within endosomes to activate Toll/interleukin-1 receptor-domain-containing adapter-inducing interferon-β (TRIF), which in turn phosphorylates IRF3, the transcription factor critical for generating type I and III interferon production. Binding of specific viral dsRNA and ssRNA motifs by RIG-I and MDA-5 triggers a signaling cascade that similarly includes TRIF but also stimulates Mitochondrial AntiViral Signaling protein (MAVS, also known as Cardif, IPS-1, or VISA) to activate IKK-related kinases (IKKε and TBK-1), which also phosphorylate IRF3 and IRF7 to trigger the production of type I and III interferons. Intracellular sensing mechanisms are initially preserved in HCV-infected hepatocytes. Type I IFN and ISGs are rapidly induced in vivo in experimentally infected chimpanzees. In humans, HCV predominantly produces a type III IFN response, predominantly with IFN-λ4 transcripts, that induces multiple ISGs that potentially could inhibit HCV viral replication by suppressing primary translation of viral RNA.
Therefore, disruption of these antiviral pathways is critical for establishment of viral persistence. Early after infection, the viral E2 and NS5A proteins both induce phosphorylation of protein kinase R (PKR) and elongation initiation factor 2a, which suppress general cellular mRNA translation without negatively impacting translation of HCV proteins. Subsequently, the NS3/4A protease cleaves MAVS, preventing its dimerization, resulting in its disassociation from mitochondrial membranes and disruption of its signaling through IKKε to activate IRF3. The NS3/4A protease may also degrade TRIF, blocking TLR3 signaling, as well as directly interacting with TBK1 and IKKε. In addition to suppressing type I and III interferon production, MAVS inactivation reduces the production of the chemokines CXCL8 and CXCL10 critical for recruitment of inflammatory cells to the liver. HCV Core also interferes with TLR3-mediated secretion of interferons, which may particularly impair TLR3-dependent type III IFN production. Furthermore, to block cellular responses to type I and III interferons that are produced, HCV Core protein upregulates SOCS3, a negative regulator of STAT phosphorylation and also directly inhibits ISGF3 activity. Thus, HCV has evolved multiple strategies not only to disrupt the generation of antiviral type I and III interferons but also to interrupt interferon-induced gene expression to maintain viral replication.
Genome-wide association studies have identified 3 polymorphisms on chromosome 19 near the IFN-λ3 gene (rs12979860, rs8099917, and ss469415590/rs368234815) that are associated with spontaneous resolution of acute infection and sensitivity to interferon-based antiviral therapy. The rs8099917 TT or rs12979860 CC polymorphisms are associated with a stronger induction of IFN-λ2 on stimulation. The ss469415590 polymorphism (TT → ΔG) causes a frameshift mutation that creates IFN-λ4, protein that binds to IFNLR to induce STAT1 and STAT2 phosphorylation, ISGF3, and ISG expression. The TT polymorphism abrogates IFN-λ4 production, which, somewhat counterintuitively, improves spontaneous resolution and antiviral treatment outcome. Paradoxically, IFN-λ4 exerts strong antiviral activity in vitro, and it remains unexplained how the presence of this highly functional antiviral protein impairs antiviral responses in HCV. In human liver, the apparent paradox appears to be relevant because intrahepatic expression of IFN-λ4 is positively rather than negatively associated with intrahepatic HCV RNA and ISG induction. Other genetic polymorphisms that impact viral sensing and ISG expression, such as the rs3747517 MDA-5 H843/T946 variant, also appear to impact the likelihood of spontaneous resolution of acute infection. IL-28B polymorphisms associated with greater clearance rates have variably been associated with more active necroinflammation and rapid fibrosis progression in chronic infection, suggesting impact of these genetic markers throughout the natural history of HCV disease.
In chronic infection, IFN-λ polymorphisms associated with viral persistence are associated with chronic upregulation of ISGs made ineffective by concurrent upregulation of inhibitory ISGs, such as USP18. Because of maximal upregulation, IFN-α therapy cannot upregulate ISGs further. Type III interferon induction of STAT1 phosphorylation does not appear to be impaired by type I interferon-induced USP18, leading to interest in the use of IFN-λ as an alternative therapeutic approach, but although better tolerated, pegylated IFN-λ to date has not shown superior antiviral efficacy. In vitro, NS3/4A protease inhibitors used as antiviral therapy can restore IFN-β secretion but at concentrations greater than 100-fold higher than the EC 50 , suggesting that restoration of type I interferon production constitutes a minor effect of protease inhibitor-based direct acting antiviral regimens.
Induction of cellular and adaptive immune responses
Once hepatocytes are productively infected with HCV, cellular defenses become activated. Type I and III interferons and stress signals from hepatocytes trigger resident DC, hepatic stellate cells (HSC), and Kupffer cells (KC) to produce cytokines, such as MIP-1α, IL-12, IL-15 and IL-18, to recruit IFN-γ-producing natural killer (NK) cells to the liver. Type I and III interferons activate liver sinusoidal endothelial cells (LSEC) to produce chemokines, such as CXCL10 and MIG, to attract T cells (reviewed in ). HCV has evolved mechanisms to disrupt several of these steps for critical efficient induction of cellular immune responses.
Dendritic Cell Dysfunction
DCs resident in tissues survey for pathogen infections, and pon detection, activate, mature, and migrate to lymphoid tissue to present antigens and induce B- and T-cell responses. Human DCs are subdivided into 3 main subtypes: CD11c+ myeloid DC (mDC1), BDCA3+ (CD141+) myeloid DC (mDC2), and CD11c-CD123+ plasmacytoid DC (pDC). Although pDC typically traffic in lymphoid organs, mDC preferentially home to peripheral tissues. On recognition of pathogens through pattern-recognition receptor (eg, TLRs), DCs increase class I and II HLA expression, upregulate costimulation ligands, and produce immunostimulatory cytokines, such as IL-12 (mDC1), IFN-λ (mDC2), and IFN-α plus IFN-λ (pDC). Circulating numbers of mDC and pDC are reduced in chronic HCV infection (reviewed in ), possibly because of enhanced homing of activated mDC1 and mDC2 to the liver.
That DC function is impaired in HCV is fairly well established, but the critical mechanisms remain controversial. Some studies indicate that HCV infection is associated with impaired DC maturation and antigen-presenting function, possibly mediated by the HCV core protein and possibly due to upregulation of indoleamine-2,3,-deoxygenase. A few studies suggest the impairment may preferentially affect pDC subset possibly by inducing pDC apoptosis. Other studies suggest that DC phagocytic function, expression of costimulation ligands, expression of class I and II HLA molecules, and cytokine function are preserved, but that antigen processing by proteasomal subunits is dysregulated. DC migration to lymphoid tissue may also be impaired because of unresponsiveness to the chemokine CCL21. Downregulation of HCV-sensing TLRs or critical adaptor molecules, such as TRIF and TRAF6, have been implicated in the reduced activation of pDC and mDC in vitro. Two key effects of impaired DC function in early HCV infection include reduced cytokine-dependent NK cell maturation and defective priming of CD4 and CD8 T cells with a resultant IL-10-secreting regulatory T-cell (Treg) phenotype.
Antiviral Effect of Nonparenchymal Liver Cells
HSC are pericytes that reside in the space of Disse between LSEC and hepatocytes, making up 5% to 8% of total human liver cells. During normal physiology, HSC store vitamin A, but once activated, HSC deposit extracellular matrix, resulting in liver. HSC themselves do not appear to be permissive to HCV infection or replication. HSC express TLR3 and may exert an antiviral effect by producing IFN-λ, which could suppress HCV replication in infected neighboring hepatocytes. However, to date, this effect has been demonstrated only in vitro. LSEC constitute approximately half of nonparenchymal liver cells. Human LSEC endocytose HCV but do not support replication. However, translated HCV RNA induces TLR7 and RIG-I-dependent production of IFN-α, IFN-β, and IFN-λ with potential antiviral effects. LSEC also may present antigen to T cells. The importance of this effect in vivo remains poorly characterized. KC are a heterogeneous group of liver-resident macrophages that line hepatic sinusoids that are critical for phagocytosing translocated bacterial products and iron reutilization. In vitro, HCV Core protein, a ligand for TLR2 present in KC, blocks TLR3-mediated production of IL-1β by macrophages, a cytokine that is thought to enhance the antiviral effects of IFN-α, suggesting that inhibition of KC activation may be critical to viral persistence. However, in chronic HCV infections, KC retain normal activation, producing high levels of IL-1β in a TLR7- and NLRP3 inflammasone-dependent manner, particularly in individuals with chronic infection and advanced fibrosis. KC also produce galectin-9, which has potent regulatory affects on HCV-specific T cells.
Innate Cellular Responses to Hepatitis C Virus Infection
NK cells are critical early antiviral effectors that kill infected target cells by secretion of perforin or through death receptor ligands, such as FasL or TRAIL, and also potently produce of IFN-γ and chemokines, such as MIP1α, MIP1β, RANTES, and granulocyte-macrophage colony-stimulating factor, needed to recruit T and B cells to infected tissues. NK cells are typically activated by stress-induced molecules, such as MHC I-like proteins (MICA, MICB, ULBP), as well as by cytokines such as IL-12, IL-15, IL-18, and IL-21. NK cell activation is intricately controlled by the balance of activating and inhibitory signals including Killer Inhibitory Receptors (KIR) (which may be activating or inhibitory despite the nomenclature) that bind HLA-C, NKG2 family receptors that bind nonclassical HLA ligands, such as HLA-E, MICA, and MICB, and activating receptors, such as NKp30, NKp44, and NKp46. Two classic subsets can be defined based on the expression of CD16 (FcγRIII, which binds IgG) and CD56; CD16 + CD56 dim (90% of NK cells) have a cytotoxic phenotype, and CD16 − CD56 bright produce greater cytokine responses. There is critical cross-talk between DCs and NK cells. DC activate NK cells by binding to NKp30 and secreting IL-12. In turn, NK cells secrete IFN-γ and TNF-α to foster DC maturation and antigen-presenting function.
Certain aspects of NK cell function in acute hepatitis C infection are genetically determined. Specifically, genetic homozygosity for a weakly inhibitor KIR gene (KIR2DL3), when coexisting with homozygosity for the strongly activating HLA-C1 gene, is strongly associated with early viral clearance. The strong impact of NK cell activation on viral clearance creates context for the evolutionary priority for HCV to dysregulate NK cell function. Highly active cytotoxic NK cells are associated with the absence of infection in highly exposed injection drug users. However, studies of acute hepatitis C patients do not universally indicate that the magnitude of NK cell cytotoxicity and IFN-γ production clearly is associated with virological outcome, with both CD16 + CD56 dim and CD16 − CD56 bright subsets showing activation. Controversy exists about the impact of chronic HCV infection of NK cell cytotoxicity. Although the frequency of peripheral NK cells appears to be decreased in chronic HCV, particularly among CD16 + CD56 dim NK cells, no difference in NK cell cytotoxicity appears to be present between chronic HCV patients and healthy donors or resolved patients.
Possible mechanisms of NK cell inhibition in chronic HCV infection in the literature include but are not limited to HCV E2 interaction with CD81, which has largely been disproven; HCV core-induced stabilization of HLA-E, which would inhibit NK cells; altered NK cell activation receptor expression; and altered NK cell cytokine production leading to impaired activation of DCs. In 2002, 2 groups showed that plate-bound HCV E2 protein interacting with CD81 reduces NK cell function in vitro. These findings have been since contradicted by studies using intact viral particles in which no inhibition occurred. In vitro, an HCV Core 35–44 peptide sequence has been shown to bind to HLA-E and stabilizes it on cell surfaces, inhibiting NK activation. The expression of HLA-E on multiple intrahepatic cell subsets including expression on HCV-infected hepatocytes was demonstrated, indicating possible in vivo relevance. Furthermore, hepatocyte HLA-E expression might skew NK cells to produce the immunosuppressive cytokines IL-10 and transforming growth factor-β (TGF-β), which inhibit DC maturation. Monocyte-derived IL-10 and TGF-β also downregulate NK cell expression of the NKG2D receptor that might reduce NK cell sensitivity to MICA and MICB on infected hepatocytes. Controversy exists about the expression of other activating receptors, such as NKp30 and NKp46, on NK cells in chronic HCV infection, with some studies showing decreases and others showing increases.
In established chronic infection, the balance of cytolytic and cytokine-producing NK cells in the liver may be associated with fibrosis progression. Intrahepatic compartmentalization of activated cytotoxic NK cells (NKG2D+, inhibitory KIR lo , CD16 + CD56 dim , NKp44 + , or TRAIL + NK cells) with reduced IFN-γ secretion correlates with liver injury. By contrast, NK cell IFN-γ production may attenuate fibrosis by HSC. Recent work suggests that contact with HCV-infected hepatocytes reduces NK cell expression of microRNA-155, which upregulates the transcription factor T-bet and the inhibitory receptor Tim-3 and was associated with reversible inhibition of NK cell IFN-γ production. Modulation of NK cell function also seems to impact response to IFN-α-based antiviral therapy with higher expression levels of inhibitory NK receptors in treatment nonresponders and increased expression of activating receptors in responders.
In addition to NK cells, natural killer T (NKT) cells are also overrepresented in liver. NKT cells are a heterogenous population of lymphocytes including invariant NKT cells that express NK cell markers, such as CD56, CD161, KIR, and NKG2 receptors, as well as a T-cell receptor. Subpopulations include invariant NKT that express a specific CD-1d-reactive T-cell receptor (most commonly Vα24Jα18/Vβ11), variant NKT cells, Vδ3 γδ T cells, and general cytolytic NKT cells (reviewed in ). The relative frequencies of NKT cells are variable in HCV-infected livers and blood. Peripheral CD56 + NKT levels are reduced early in acute HCV infection, but whether this is due to compartmentalization to the liver is not known, an effect suggested by the association of intrahepatic activated NKT cells and spontaneous recovery. In chronic infection, there appears to be a regulatory effect of NKT cells that prime naive antigen-specific CD8 + T cells to produce IL-10.
Adaptive Cellular Response to Hepatitis C Virus Infection
After activation, mDC and pDC migrate to lymphoid tissue to stimulate the generation of antigen-specific B- and T-cell responses. Early adaptive immune responses are critical in the outcome of viral infections. CD8 + T cells are the immune effectors that directly eliminate virus-infected cells by both cytolytic and noncytolytic mechanisms, whereas CD4 + T cells play a key regulatory role, providing help for CD8 + T cells and B cells.
The strength and scope of HCV-specific T-cell response are strongly associated with the outcome of acute HCV infection. In humans with acute hepatitis C, sustained vigorous and multispecific CD4 + and CD8 + IFN-γ + T-cell responses to HCV (particularly to nonstructural antigens) in peripheral blood are associated with resolution of acute infection, whereas weak, focused, or transient responses are associated with viral persistence. In experimentally infected chimpanzees, HCV-specific T-cell responses in the liver correlate with virological outcome, validating the observations made with peripheral blood in patients. Cell depletion experiments in chimpanzees confirm that both CD4 + and CD8 + T cells are needed for efficient viral clearance. During very early phases of acute hepatitis infection, the capacity of CD4 + T cells to proliferate in response to viral antigens is associated with conversion of hyporeactive “stunned” HCV-specific CD8 + T cells into strong antiviral effectors that can be cytotoxic to infected hepatocytes.
The role of HCV-specific effector T cells in the long-term outcome of chronic infection is less well defined. The detection of, albeit weak, peripheral HCV-specific CD4 + T-cell IFN-γ responses has been associated with a more benign clinical course. No consistent link between intrahepatic HCV-specific CD4 + and CD8 + T-cell IFN-γ frequencies and long-term clinical outcomes has been shown. However, circumstantial evidence for the importance of effector T cells in control of HCV-related liver disease can be found in the setting of generalized T-cell defects (eg, HIV/HCV coinfection, chronic steroid use, posttransplant immunosuppression) in which immune dysregulation leads to accelerated liver disease progression and high viral titers.
Failure of antiviral T cells to control initial HCV infection is multifactorial, likely resulting from impaired priming of T cells by DC (discussed earlier), aberrant T-cell priming by intrahepatic antigen-presenting cells (LSEC, hepatocytes, NKT), viral escape mutation, induction of various Treg subsets, and T-cell anergy. Specific viral sequences in founder viruses may have varying degrees of immunogenicity, and certain HLA alleles are more or less likely to induce sterilizing immune responses. Presentation of epitopes from viral escape variants may antagonize HCV-specific T-cell responses. Furthermore, HCV core may suppress T-cell activation through the C1q complement receptor. The induction of multiple subtypes of Treg and antigen-induced expression of inhibitory costimulation receptors are critical additional steps required for viral persistence.
Treg, typically defined by expression of the transcription factor foxp3 and high expression of the IL-2 receptor α chain (CD25), relevant in hepatitis C infection include natural CD304 + Tregs (nTreg) specific for self-epitopes and induced Tregs derived from virus-specific CD4 + CD25 − effector T cells. Activated Tregs suppress effector T cells in a contact-dependent manner within target tissues. The generation of nTregs sequence homology for self-epitopes present in the HCV Core and p7 antigens. In vitro, CD4 + T cells cocultured with HCV-infected hepatocyte cell lines develop a Treg phenotype with increased expression of foxp3, CD25, CTLA-4, and TGF-β. High-level expression of a viral antigen in hepatocytes may also be a critical factor predisposing to the development of Tregs. The overall frequency of Tregs does not differ in acute resolving or persisting HCV infection, but the suppressive capacity of Tregs increases over time in persistent acute infection while decreasing in resolving infection. In chronic infection, there is not only increased circulating CD4 + CD25 + Tregs but also significant CD4 + foxp3 + Treg infiltration into the liver with associated suppression of necroinflammation and fibrosis. Several mouse models of acute and chronic hepatitis have provided strong evidence that Tregs play a fundamental role in dampening the potentially deleterious impact of activated effector T cells in the liver.
HCV-specific IL-10-producing Tr1 T cells, typically not expressing foxp3 or high-level CD25, comprise an additional regulatory cell population important for HCV pathogenesis. Although IL-10 is produced by many cell types, including DCs, macrophages, monocytes, NK, and NKT cells, IL-10 + foxp3 − CD4 + T cells appear to be an important source of IL-10 and TGF-β, directly inhibiting effector T-cell function in both IL-10-dependent and IL-10-independent fashion. In vitro, HCV proteins directly induce virus-specific T-cell production of IL-10 in both CD4 + and CD8 + T-cell subsets. In acute infection, early skewing of antiviral T cells to produce IL-10, possibly influenced by polymorphisms in the IL-10 promoter, is associated with chronic evolution. Viral mutation under immune selection pressure may also reprogram T cells to produce IL-10. Because of its anti-inflammatory effects, exogenous administration of IL-10 in humans reduces hepatic fibrosis. The frequency of CD8 + IL-10 + T cells in the liver also inversely correlates with inflammation, suggesting that T-cell IL-10 production may be a critical negative feedback to prevent rapid liver injury in chronic infection.
In chronic infection, prolonged antigenic stimulation leads to the upregulation of several inhibitory coreceptors on antigen-specific CD8 + T cells that individually or in combination generate a state of functional hyporeactivity, termed anergy. The inhibitory receptors best characterized for this effect include programmed death-1 (PD-1), cytotoxic T-lymphocyte associated protein-4 (CTLA-4), T-cell immunoglobulin and mucin 3 engagement of high-mobility group box 1, NK cell receptor 2B4 (CD244), lymphocyte associated gene-3, Killer cell lectin-like receptor subfamily G member 1, and CD160.
PD-1 is 55-kDa glycoprotein member of the CD28 superfamily that contains an immunoreceptor tyrosine-based inhibitory motif and immunoreceptor switch motif that phosphorylate the kinase SHP-2, which then dephosphorylates various signal transduction kinases involved in T-cell receptor induction of IL-2 and proliferation. The ligand for PD-1, PD-L1, is expressed on intrahepatic mDC, LSEC, and KC. CTLA-4 is an immunoglobulin-like receptor that competes with CD28 for binding of CD80 and CD86, thereby blocking T-cell costimulation. PD-1 and CTLA-4 become highly expressed on HCV-specific CD8 + T cells and CD4 + T cells early in acute HCV infection, remaining elevated in persistent infection but normalizing after viral clearance. In chronic infection, HCV-specific memory CD8 + T cells particularly within the liver express high levels of PD-1, impaired effector cytokines production, and low levels of cytolytic granules. Early studies indicated that single blockade of PD-1/PD-L1 interactions ex vivo augments the expansion of peripheral CD8 + T-cell expansion. However, intrahepatic HCV-specific CD8 + T cells from patients with cirrhosis express high levels of other costimulation inhibitory receptors such as CTLA-4 and require multiple pathway blockade to restore antigen-specific responses in some patients. More recent studies suggest that among peripheral HCV-specific CD8 + T cells in individual patients, various combinations of inhibitory receptors may be expressed and that responses to blockade of any single inhibitor is highly variable ; indeed, a 4-pathway blockade was required to improve HCV-specific CD8 + T-cell function in greater than half of the patient samples in one study. Other studies suggest that the anergic state is irreversible, even after curing infection through antiviral therapy and that residual functional CD8 + T cells detected ex vivo are specific for viral sequence not expressed by circulating virus. Therapeutically, the use of anti-PD-1 antibody appeared to cause significant reduction of viral loads in one-third of treated chimpanzees and a similar number of humans ; in light of the relative inefficacy of single blockade in ex vivo experiments and the rapid evolution of direct acting antivirals, the further development of this treatment strategy is unknown. Nonetheless, induction of T-cell anergy is thought to play a critical role in preventing sterilizing antiviral CD8 + T-cell responses in both acute and chronic HCV infection.
The B-cell-mediated antibody response to HCV can be detected within 6 to 8 weeks of inoculation. Neutralizing antibodies, which interfere with viral envelope binding to targets such as LDLR, SRBI, CD81, and claudin-1, in early acute infection are associated with resolution in some but not all studies. However, chimpanzee cross-challenge experiments and human series show that HCV-specific antibodies do not universally mediate protection. Possible reasons for the lack of antibody-mediated protection include (1) the mutable quasi-species nature of HCV with rapid selection of antibody escape variants ; (2) intrinsic sequence-specific variability of the sensitivity of E1E2 proteins to neutralization ; and (3) paradoxic facilitation of viral entry by sensitivity of E1E2 protein. In up to 40% of patients with spontaneous viral clearance, HCV antibody titers may wane after 2 to 3 decades. In persistent infection, novel B-cell clones are continuously stimulated to respond to evolving viral mutations. In addition, interactions between the HCV E2 envelope protein and B-cell CD81, an activating tetraspanin coreceptor, drive antigen-independent polyclonal B-cell stimulation, predisposing to B-cell lymphoproliferative disorders. Despite chronic activation of virus-specific and non-virus-specific B cells, memory B cells do not accumulate in chronically infected patients for reasons yet to be clearly defined. As seen with T cells, chronic viral infection creates an anergic state in some HCV-specific B cells, phenotypically defined as CD27 + CD27 −/lo tissue-like memory B cells with impaired proliferation with unclear clinical significance. A clear pathogenic role for B cells in liver disease progression in chronic HCV infection has not as yet been defined.
Summary
The establishment of persistent infection, resistance to interferon-based therapy, and progression of fibrosis are tightly linked with immune dysregulation induced by specific hepatitis C viral proteins or by rapid viral mutation. Genetic polymorphisms that alter either cytokine responses, antigen-independent stimulation of innate immune cells, or antigen presentation in part determine the susceptibility of an individual human host to initial infection, and the impact on the liver of a persistent infection. Other host factors, some quantifiable, such as age, gender, alcohol use, and pre-existing immunologic defects, and others poorly quantifiable, shape the complex dynamic host-virus interactions that determine permissiveness to chronic infection and disease progression rates. Critical events for the establishment of persistent infection include the disruption of type I and III interferon induction and signaling, interference with innate cellular activation, impairment and skewing of antigen-presentation to B and T cells, induction of various Treg T cell subsets, and functional inhibition of antigen-specific T- and B-cell responses. After initial failure to control infection, these processes control the magnitude of necroinflammation and resultant fibrosis progression. The complexity of interactions makes it unlikely that any therapeutic modality aimed at any single component will have universal efficacy, thus defining the critical nodes in these networks suitable for intervention remains an important endeavor despite the development of highly effective, oral therapy for chronic hepatitis C.
Intrahepatocytic sensing and signaling
HCV is a highly mutable, hepatotropic, enveloped single-stranded RNA virus of the Flaviviridae family. The 9.5-kB genome encodes for a single polyprotein of 3011 amino acids flanked by 5′ and 3′ noncoding regions essential for viral replication. The viral polyprotein is translated in toto and then processed by host cell- and virus-encoded proteases into structural (Core, E1, E2) and nonstructural proteins (p7, NS2, NS3, NS4A, NS4B, NS5A, NS5B). The Core protein forms the viral nucleocapsid. E1 and E2 are the viral envelope glycoproteins that interact with various surface receptors, including claudin-1, CD81, DC-SIGN, scavenger receptor B I (SR-BI), and the LDL receptor, to mediate viral entry into target cells. The remainder of the nonstructural proteins creates a membrane-associated viral replication complex critical for establishment for persistent, and along with structural proteins, modulates host cell function to disrupt intracellular and extracellular antiviral pathways ( Table 1 ).
Core | Activation of PKR |
Inhibition of TLR3-mediated secretion of type I and III interferon | |
Inhibition of STAT1 activation | |
Inhibition of ISGFR3 nuclear translocation | |
Impairment of dendritic cell maturation and antigen presentation | |
Blockade of IL-1β production in macrophages | |
Stabilization of HLA-E | |
C1q-mediated T-cell inhibition | |
E2 | Activation of PKR and elongation initiation factor 2a (eIF2a) |
Stimulation of CD81 on B cells | |
NS2 | Inhibition of TBK1 and IKKe |
NS3/4A | Cleavage of MAVS |
Inhibition of TBK1 and IKKe | |
Cleavage of TRIF | |
Suppression of CXCL8 and CXCL10 chemokines | |
NS5A | Activation of PKR |
NS5B | Viral mutations leading to escape or inhibition of T-cell function |
The induction of type I (IFN-α and IFN-β), II (IFN-γ), and III (interleukin [IL]-28A/IFN-λ2, IL-28B/IFN-λ3, IL-29/IFN-λ1, and IFN-λ4) interferons is critical for host-defense against intracellular pathogens. Type I, II, and III IFNs signals in autocrine and paracrine manners through their respective heterodimeric receptors (IFNAR, IFNGR, IFNLR) to phosphorylate STAT-1 and STAT-2, leading to the formation of the transcription factor ISGF3 (consisting of pSTAT-1, pSTAT-2, and Interferon Response Factor [IRF] 9) that translocates to the nucleus to modulate the production of hundreds of IFN-stimulated antiviral genes (ISGs). Specific ISGs suppress viral replication and sensitize infected cells to apoptosis. Critical to the development of adaptive immune responses, type I interferons also stimulate immunoproteasome formation critical for presentation of antigen by hepatocytes to CD8 T cells. Although IFNAR and IFNGR expression is ubiquitous, the heterodimeric IFNLR (consisting of IFN-λR1 and IL-10R2 chains) is restricted to epithelial cells, hepatocytes, and dendritic cells (DCs). Although there is significant overlap in the number and types of genes induced by type I, II, and III interferons, IFN-α and IFN-λ do have subtle differences in gene expression profiles and kinetics. Notably, the permissiveness of hepatocyte cell lines to support HCV infection in vitro appears critically dependent on defects in type I and III interferon signaling.
After viral entry and uncoating, hepatocytes sense intracellular HCV infection to generate type I and III interferons both by toll-like receptors (TLRs) and by retinoic-acid-inducible gene-like receptors, a family of proteins that includes retinoic acid-inducible gene I (RIG-I), melanoma differentiation-associated gene 5 (MDA-5), and laboratory of genetics and physiology gene 2 (Lgp2), in the cytoplasm. TLR3 recognizes double-stranded RNA replication intermediates on the cell membrane or within endosomes to activate Toll/interleukin-1 receptor-domain-containing adapter-inducing interferon-β (TRIF), which in turn phosphorylates IRF3, the transcription factor critical for generating type I and III interferon production. Binding of specific viral dsRNA and ssRNA motifs by RIG-I and MDA-5 triggers a signaling cascade that similarly includes TRIF but also stimulates Mitochondrial AntiViral Signaling protein (MAVS, also known as Cardif, IPS-1, or VISA) to activate IKK-related kinases (IKKε and TBK-1), which also phosphorylate IRF3 and IRF7 to trigger the production of type I and III interferons. Intracellular sensing mechanisms are initially preserved in HCV-infected hepatocytes. Type I IFN and ISGs are rapidly induced in vivo in experimentally infected chimpanzees. In humans, HCV predominantly produces a type III IFN response, predominantly with IFN-λ4 transcripts, that induces multiple ISGs that potentially could inhibit HCV viral replication by suppressing primary translation of viral RNA.
Therefore, disruption of these antiviral pathways is critical for establishment of viral persistence. Early after infection, the viral E2 and NS5A proteins both induce phosphorylation of protein kinase R (PKR) and elongation initiation factor 2a, which suppress general cellular mRNA translation without negatively impacting translation of HCV proteins. Subsequently, the NS3/4A protease cleaves MAVS, preventing its dimerization, resulting in its disassociation from mitochondrial membranes and disruption of its signaling through IKKε to activate IRF3. The NS3/4A protease may also degrade TRIF, blocking TLR3 signaling, as well as directly interacting with TBK1 and IKKε. In addition to suppressing type I and III interferon production, MAVS inactivation reduces the production of the chemokines CXCL8 and CXCL10 critical for recruitment of inflammatory cells to the liver. HCV Core also interferes with TLR3-mediated secretion of interferons, which may particularly impair TLR3-dependent type III IFN production. Furthermore, to block cellular responses to type I and III interferons that are produced, HCV Core protein upregulates SOCS3, a negative regulator of STAT phosphorylation and also directly inhibits ISGF3 activity. Thus, HCV has evolved multiple strategies not only to disrupt the generation of antiviral type I and III interferons but also to interrupt interferon-induced gene expression to maintain viral replication.
Genome-wide association studies have identified 3 polymorphisms on chromosome 19 near the IFN-λ3 gene (rs12979860, rs8099917, and ss469415590/rs368234815) that are associated with spontaneous resolution of acute infection and sensitivity to interferon-based antiviral therapy. The rs8099917 TT or rs12979860 CC polymorphisms are associated with a stronger induction of IFN-λ2 on stimulation. The ss469415590 polymorphism (TT → ΔG) causes a frameshift mutation that creates IFN-λ4, protein that binds to IFNLR to induce STAT1 and STAT2 phosphorylation, ISGF3, and ISG expression. The TT polymorphism abrogates IFN-λ4 production, which, somewhat counterintuitively, improves spontaneous resolution and antiviral treatment outcome. Paradoxically, IFN-λ4 exerts strong antiviral activity in vitro, and it remains unexplained how the presence of this highly functional antiviral protein impairs antiviral responses in HCV. In human liver, the apparent paradox appears to be relevant because intrahepatic expression of IFN-λ4 is positively rather than negatively associated with intrahepatic HCV RNA and ISG induction. Other genetic polymorphisms that impact viral sensing and ISG expression, such as the rs3747517 MDA-5 H843/T946 variant, also appear to impact the likelihood of spontaneous resolution of acute infection. IL-28B polymorphisms associated with greater clearance rates have variably been associated with more active necroinflammation and rapid fibrosis progression in chronic infection, suggesting impact of these genetic markers throughout the natural history of HCV disease.
In chronic infection, IFN-λ polymorphisms associated with viral persistence are associated with chronic upregulation of ISGs made ineffective by concurrent upregulation of inhibitory ISGs, such as USP18. Because of maximal upregulation, IFN-α therapy cannot upregulate ISGs further. Type III interferon induction of STAT1 phosphorylation does not appear to be impaired by type I interferon-induced USP18, leading to interest in the use of IFN-λ as an alternative therapeutic approach, but although better tolerated, pegylated IFN-λ to date has not shown superior antiviral efficacy. In vitro, NS3/4A protease inhibitors used as antiviral therapy can restore IFN-β secretion but at concentrations greater than 100-fold higher than the EC 50 , suggesting that restoration of type I interferon production constitutes a minor effect of protease inhibitor-based direct acting antiviral regimens.
References
- 1. Lavanchy D.: The global burden of hepatitis C. Liver Int 2009; 29: pp. 74-81
- 2. Alter H.J.: To C or not to C: these are the questions. Blood 1995; 85: pp. 1681-1695
- 3. Major M.E., and Feinstone S.M.: The molecular virology of hepatitis C. Hepatology 1997; 25: pp. 1527-1538
- 4. Houghton M., Weiner A., Han J., et al: Molecular biology of the hepatitis C viruses: implications for diagnosis, development and control of viral disease. Hepatology 1991; 14: pp. 381-388
- 5. Pileri P., Uematsu Y., Campagnoli S., et al: Binding of hepatitis C virus to CD81. Science 1998; 282: pp. 938-941
- 6. Hsu M., Zhang J., Flint M., et al: Hepatitis C virus glycoproteins mediate pH-dependent cell entry of pseudotyped retroviral particles. Proc Natl Acad Sci U S A 2003; 100: pp. 7271-7276
- 7. Wunschmann S., Medh J.D., Klinzmann D., et al: Characterization of hepatitis C virus (HCV) and HCV E2 interactions with CD81 and the low-density lipoprotein receptor. J Virol 2000; 74: pp. 10055-10062
- 8. Lozach P.Y., Lortat-Jacob H., de Lacroix de Lavalette A., et al: DC-SIGN and L-SIGN are high affinity binding receptors for hepatitis C virus glycoprotein E2. J Biol Chem 2003; 278: pp. 20358-20366
- 9. Pohlmann S., Zhang J., Baribaud F., et al: Hepatitis C virus glycoproteins interact with DC-SIGN and DC-SIGNR. J Virol 2003; 77: pp. 4070-4080
- 10. McKeating J.A., Zhang L.Q., Logvinoff C., et al: Diverse hepatitis C virus glycoproteins mediate viral infection in a CD81-dependent manner. J Virol 2004; 78: pp. 8496-8505
- 11. Evans M.J., von Hahn T., Tscherne D.M., et al: Claudin-1 is a hepatitis C virus co-receptor required for a late step in entry. Nature 2007; 446: pp. 801-805
- 12. Stetson D.B., and Medzhitov R.: Type I interferons in host defense. Immunity 2006; 25: pp. 373-381
- 13. Metz P., Dazert E., Ruggieri A., et al: Identification of type I and type II interferon-induced effectors controlling hepatitis C virus replication. Hepatology 2012; 56: pp. 2082-2093
- 14. Shin E.C., Seifert U., Kato T., et al: Virus-induced type I IFN stimulates generation of immunoproteasomes at the site of infection. J Clin Invest 2006; 116: pp. 3006-3014
- 15. Yoshio S., Kanto T., Kuroda S., et al: Human blood dendritic cell antigen 3 (BDCA3)(+) dendritic cells are a potent producer of interferon-lambda in response to hepatitis C virus. Hepatology 2013; 57: pp. 1705-1715
- 16. Marcello T., Grakoui A., Barba-Spaeth G., et al: Interferons alpha and lambda inhibit hepatitis C virus replication with distinct signal transduction and gene regulation kinetics. Gastroenterology 2006; 131: pp. 1887-1898
- 17. Kohli A., Zhang X., Yang J., et al: Distinct and overlapping genomic profiles and antiviral effects of interferon-lambda and -alpha on HCV-infected and noninfected hepatoma cells. J Viral Hepat 2012; 19: pp. 843-853
- 18. Dickensheets H., Sheikh F., Park O., et al: Interferon-lambda (IFN-lambda) induces signal transduction and gene expression in human hepatocytes, but not in lymphocytes or monocytes. J Leukoc Biol 2013; 93: pp. 377-385
- 19. Sumpter R., Loo Y.M., Foy E., et al: Regulating intracellular antiviral defense and permissiveness to hepatitis C virus RNA replication through a cellular RNA helicase, RIG-I. J Virol 2005; 79: pp. 2689-2699
- 20. Israelow B., Narbus C.M., Sourisseau M., et al: HepG2 cells mount an effective antiviral interferon-lambda based innate immune response to hepatitis C virus infection. Hepatology 2014; 60: pp. 1170-1179
- 21. Foy E., Li K., Sumpter R., et al: Control of antiviral defenses through hepatitis C virus disruption of retinoic acid-inducible gene-I signaling. Proc Natl Acad Sci U S A 2005; 102: pp. 2986-2991
- 22. Saito T., and Gale M.: Principles of intracellular viral recognition. Curr Opin Immunol 2007; 19: pp. 17-23
- 23. Saito T., Owen D.M., Jiang F., et al: Innate immunity induced by composition-dependent RIG-I recognition of hepatitis C virus RNA. Nature 2008; 454: pp. 523-527
- 24. Su A.I., Pezacki J.P., Wodicka L., et al: Genomic analysis of the host response to hepatitis C virus infection. Proc Natl Acad Sci U S A 2002; 99: pp. 15669-15674
- 25. Thomas E., Gonzalez V.D., Li Q., et al: HCV infection induces a unique hepatic innate immune response associated with robust production of type III interferons. Gastroenterology 2012; 142: pp. 978-988
- 26. Amanzada A., Kopp W., Spengler U., et al: Interferon-lambda4 (IFNL4) transcript expression in human liver tissue samples. PLoS One 2013; 8: pp. e84026
- 27. Terczynska-Dyla E., Bibert S., Duong F.H., et al: Reduced IFNlambda4 activity is associated with improved HCV clearance and reduced expression of interferon-stimulated genes. Nat Commun 2014; 5: pp. 5699
- 28. Schoggins J.W., MacDuff D.A., Imanaka N., et al: Pan-viral specificity of IFN-induced genes reveals new roles for cGAS in innate immunity. Nature 2014; 505: pp. 691-695
- 29. Gale M., Blakely C.M., Kwieciszewski B., et al: Control of PKR protein kinase by hepatitis C virus nonstructural 5A protein: molecular mechanisms of kinase regulation. Mol Cell Biol 1998; 18: pp. 5208-5218
- 30. Taylor D.R., Shi S.T., Romano P.R., et al: Inhibition of the interferon-inducible protein kinase PKR by HCV E2 protein. Science 1999; 285: pp. 107-110
- 31. Arnaud N., Dabo S., Maillard P., et al: Hepatitis C virus controls interferon production through PKR activation. PLoS One 2010; 5: pp. e10575
- 32. Meylan E., Curran J., Hofmann K., et al: Cardif is an adaptor protein in the RIG-I antiviral pathway and is targeted by hepatitis C virus. Nature 2005; 437: pp. 1167-1172
- 33. Breiman A., Grandvaux N., Lin R., et al: Inhibition of RIG-I-dependent signaling to the interferon pathway during hepatitis C virus expression and restoration of signaling by IKKepsilon. J Virol 2005; 79: pp. 3969-3978
- 34. Loo Y.M., Owen D.M., Li K., et al: Viral and therapeutic control of IFN-beta promoter stimulator 1 during hepatitis C virus infection. Proc Natl Acad Sci U S A 2006; 103: pp. 6001-6006
- 35. Lin R., Lacoste J., Nakhaei P., et al: Dissociation of a MAVS/IPS-1/VISA/Cardif-IKKepsilon molecular complex from the mitochondrial outer membrane by hepatitis C virus NS3-4A proteolytic cleavage. J Virol 2006; 80: pp. 6072-6083
- 36. Cheng G., Zhong J., and Chisari F.V.: Inhibition of dsRNA-induced signaling in hepatitis C virus-infected cells by NS3 protease-dependent and -independent mechanisms. Proc Natl Acad Sci U S A 2006; 103: pp. 8499-8504
- 37. Tasaka M., Sakamoto N., Itakura Y., et al: Hepatitis C virus non-structural proteins responsible for suppression of the RIG-I/Cardif-induced interferon response. J Gen Virol 2007; 88: pp. 3323-3333
- 38. Baril M., Racine M.E., Penin F., et al: MAVS dimer is a crucial signaling component of innate immunity and the target of hepatitis C virus NS3/4A protease. J Virol 2009; 83: pp. 1299-1311
- 39. Ding Q., Huang B., Lu J., et al: Hepatitis C virus NS3/4A protease blocks IL-28 production. Eur J Immunol 2012; 42: pp. 2374-2382
- 40. Li K., Foy E., Ferreon J.C., et al: Immune evasion by hepatitis C virus NS3/4A protease-mediated cleavage of the Toll-like receptor 3 adaptor protein TRIF. Proc Natl Acad Sci U S A 2005; 102: pp. 2992-2997
- 41. Otsuka M., Kato N., Moriyama M., et al: Interaction between the HCV NS3 protein and the host TBK1 protein leads to inhibition of cellular antiviral responses. Hepatology 2005; 41: pp. 1004-1012
- 42. Wagoner J., Austin M., Green J., et al: Regulation of CXCL-8 (interleukin-8) induction by double-stranded RNA signaling pathways during hepatitis C virus infection. J Virol 2007; 81: pp. 309-318
- 43. Tu Z., Pierce R.H., Kurtis J., et al: Hepatitis C virus core protein subverts the antiviral activities of human Kupffer cells. Gastroenterology 2010; 138: pp. 305-314
- 44. Ebihara T., Shingai M., Matsumoto M., et al: Hepatitis C virus-infected hepatocytes extrinsically modulate dendritic cell maturation to activate T cells and natural killer cells. Hepatology 2008; 48: pp. 48-58
- 45. Okamoto M., Oshiumi H., Azuma M., et al: IPS-1 is essential for type III IFN production by hepatocytes and dendritic cells in response to hepatitis C virus infection. J Immunol 2014; 192: pp. 2770-2777
- 46. Bode J.G., Ludwig S., Ehrhardt C., et al: IFN-alpha antagonistic activity of HCV core protein involves induction of suppressor of cytokine signaling-3. FASEB J 2003; 17: pp. 488-490
- 47. Melen K., Fagerlund R., Nyqvist M., et al: Expression of hepatitis C virus core protein inhibits interferon-induced nuclear import of STATs. J Med Virol 2004; 73: pp. 536-547
- 48. de Lucas S., Bartolome J., and Carreno V.: Hepatitis C virus core protein down-regulates transcription of interferon-induced antiviral genes. J Infect Dis 2005; 191: pp. 93-99
- 49. Lin W., Kim S.S., Yeung E., et al: Hepatitis C virus core protein blocks interferon signaling by interaction with the STAT1 SH2 domain. J Virol 2006; 80: pp. 9226-9235
- 50. Thomas D.L., Thio C.L., Martin M.P., et al: Genetic variation in IL28B and spontaneous clearance of hepatitis C virus. Nature 2009; 461: pp. 798-801
- 51. Rauch A., Kutalik Z., Descombes P., et al: Genetic variation in IL28B is associated with chronic hepatitis C and treatment failure: a genome-wide association study. Gastroenterology 2010; 138: pp. 1338-1345
- 52. Hajarizadeh B., Grady B., Page K., et al: Patterns of hepatitis C virus RNA levels during acute infection: the InC3 study. PLoS One 2015; 10: pp. e0122232
- 53. Ge D., Fellay J., Thompson A.J., et al: Genetic variation in IL28B predicts hepatitis C treatment-induced viral clearance. Nature 2009; 461: pp. 399-401
- 54. Suppiah V., Moldovan M., Ahlenstiel G., et al: IL28B is associated with response to chronic hepatitis C interferon-alpha and ribavirin therapy. Nat Genet 2009; 41: pp. 1100-1104
- 55. Tanaka Y., Nishida N., Sugiyama M., et al: Genome-wide association of IL28B with response to pegylated interferon-alpha and ribavirin therapy for chronic hepatitis C. Nat Genet 2009; 41: pp. 1105-1109
- 56. Prokunina-Olsson L., Muchmore B., Tang W., et al: A variant upstream of IFNL3 (IL28B) creating a new interferon gene IFNL4 is associated with impaired clearance of hepatitis C virus. Nat Genet 2013; 45: pp. 164-171
- 57. Real L.M., Neukam K., Herrero R., et al: IFNL4 ss469415590 variant shows similar performance to rs12979860 as predictor of response to treatment against Hepatitis C Virus genotype 1 or 4 in Caucasians. PLoS One 2014; 9: pp. e95515
- 58. Stattermayer A.F., Strassl R., Maieron A., et al: Polymorphisms of interferon-lambda4 and IL28B—effects on treatment response to interferon/ribavirin in patients with chronic hepatitis C. Aliment Pharmacol Ther 2014; 39: pp. 104-111
- 59. Susser S., Herrmann E., Lange C., et al: Predictive value of interferon-lambda gene polymorphisms for treatment response in chronic hepatitis C. PLoS One 2014; 9: pp. e112592
- 60. Bibert S., Roger T., Calandra T., et al: IL28B expression depends on a novel TT/-G polymorphism which improves HCV clearance prediction. J Exp Med 2013; 210: pp. 1109-1116
- 61. Hamming O.J., Terczynska-Dyla E., Vieyres G., et al: Interferon lambda 4 signals via the IFNlambda receptor to regulate antiviral activity against HCV and coronaviruses. EMBO J 2013; 32: pp. 3055-3065
- 62. Lu Y.F., Goldstein D.B., Urban T.J., et al: Interferon-lambda4 is a cell-autonomous type III interferon associated with pre-treatment hepatitis C virus burden. Virology 2015; 476: pp. 334-340
- 63. Hoffmann F.S., Schmidt A., Dittmann Chevillotte M., et al: Polymorphisms in melanoma differentiation-associated gene 5 link protein function to clearance of hepatitis C virus. Hepatology 2015; 61: pp. 460-470
- 64. Marabita F., Aghemo A., De Nicola S., et al: Genetic variation in the interleukin-28B gene is not associated with fibrosis progression in patients with chronic hepatitis C and known date of infection. Hepatology 2011; 54: pp. 1127-1134
- 65. Bochud P.Y., Bibert S., Kutalik Z., et al: IL28B alleles associated with poor hepatitis C virus (HCV) clearance protect against inflammation and fibrosis in patients infected with non-1 HCV genotypes. Hepatology 2012; 55: pp. 384-394
- 66. Noureddin M., Wright E.C., Alter H.J., et al: Association of IL28B genotype with fibrosis progression and clinical outcomes in patients with chronic hepatitis C: a longitudinal analysis. Hepatology 2013; 58: pp. 1548-1557
- 67. Eslam M., Leung R., Romero-Gomez M., et al: IFNL3 polymorphisms predict response to therapy in chronic hepatitis C genotype 2/3 infection. J Hepatol 2014; 61: pp. 235-241
- 68. Randall G., Chen L., Panis M., et al: Silencing of USP18 potentiates the antiviral activity of interferon against hepatitis C virus infection. Gastroenterology 2006; 131: pp. 1584-1591
- 69. Urban T.J., Thompson A.J., Bradrick S.S., et al: IL28B genotype is associated with differential expression of intrahepatic interferon-stimulated genes in patients with chronic hepatitis C. Hepatology 2010; 52: pp. 1888-1896
- 70. Makowska Z., Duong F.H., Trincucci G., et al: Interferon-beta and interferon-lambda signaling is not affected by interferon-induced refractoriness to interferon-alpha in vivo. Hepatology 2011; 53: pp. 1154-1163
- 71. Sarasin-Filipowicz M., Oakeley E.J., Duong F.H., et al: Interferon signaling and treatment outcome in chronic hepatitis C. Proc Natl Acad Sci U S A 2008; 105: pp. 7034-7039
- 72. Feld J.J., Lutchman G.A., Heller T., et al: Ribavirin improves early responses to peginterferon through improved interferon signaling. Gastroenterology 2010; 139: pp. 154-162.e4
- 73. Bigger C.B., Brasky K.M., and Lanford R.E.: DNA microarray analysis of chimpanzee liver during acute resolving hepatitis C virus infection. J Virol 2001; 75: pp. 7059-7066
- 74. Bigger C.B., Guerra B., Brasky K.M., et al: Intrahepatic gene expression during chronic hepatitis C virus infection in chimpanzees. J Virol 2004; 78: pp. 13779-13792
- 75. Chen L., Borozan I., Feld J., et al: Hepatic gene expression discriminates responders and nonresponders in treatment of chronic hepatitis C viral infection. Gastroenterology 2005; 128: pp. 1437-1444
- 76. Feld J.J., Nanda S., Huang Y., et al: Hepatic gene expression during treatment with peginterferon and ribavirin: identifying molecular pathways for treatment response. Hepatology 2007; 46: pp. 1548-1563
- 77. Muir A.J., Shiffman M.L., Zaman A., et al: Phase 1b study of pegylated interferon lambda 1 with or without ribavirin in patients with chronic genotype 1 hepatitis C virus infection. Hepatology 2010; 52: pp. 822-832
- 78. Liang Y., Ishida H., Lenz O., et al: Antiviral suppression vs restoration of RIG-I signaling by hepatitis C protease and polymerase inhibitors. Gastroenterology 2008; 135: pp. 1710-1718.e2
- 79. Ahmad A., and Alvarez F.: Role of NK and NKT cells in the immunopathogenesis of HCV-induced hepatitis. J Leukoc Biol 2004; 76: pp. 743-759
- 80. Velazquez V.M., Hon H., Ibegbu C., et al: Hepatic enrichment and activation of myeloid dendritic cells during chronic hepatitis C virus infection. Hepatology 2012; 56: pp. 2071-2081
- 81. Takahashi K., Asabe S., Wieland S., et al: Plasmacytoid dendritic cells sense hepatitis C virus-infected cells, produce interferon, and inhibit infection. Proc Natl Acad Sci U S A 2010; 107: pp. 7431-7436
- 82. Grabski E., Wappler I., Pfaender S., et al: Efficient virus assembly, but not infectivity, determines the magnitude of hepatitis C virus-induced interferon alpha responses of plasmacytoid dendritic cells. J Virol 2015; 89: pp. 3200-3208
- 83. Zhang S., Kodys K., Babcock G.J., et al: CD81/CD9 tetraspanins aid plasmacytoid dendritic cells in recognition of hepatitis C virus-infected cells and induction of interferon-alpha. Hepatology 2013; 58: pp. 940-949
- 84. Zhang S., Kodys K., Li K., et al: Human type 2 myeloid dendritic cells produce interferon-lambda and amplify interferon-alpha in response to hepatitis C virus infection. Gastroenterology 2013; 144: pp. 414-425.e7
- 85. Leone P., Di Tacchio M., Berardi S., et al: Dendritic cell maturation in HCV infection: altered regulation of MHC class I antigen processing-presenting machinery. J Hepatol 2014; 61: pp. 242-251
- 86. Murata K., Sugiyama M., Kimura T., et al: Ex vivo induction of IFN-lambda3 by a TLR7 agonist determines response to Peg-IFN/ribavirin therapy in chronic hepatitis C patients. J Gastroenterol 2014; 49: pp. 126-137
- 87. Marukian S., Andrus L., Sheahan T.P., et al: Hepatitis C virus induces interferon-lambda and interferon-stimulated genes in primary liver cultures. Hepatology 2011; 54: pp. 1913-1923
- 88. Ryan E.J., and O’Farrelly C.: The affect of chronic hepatitis C infection on dendritic cell function: a summary of the experimental evidence. J Viral Hepat 2011; 18: pp. 601-607
- 89. Auffermann-Gretzinger S., Keeffe E.B., and Levy S.: Impaired dendritic cell maturation in patients with chronic, but not resolved, hepatitis C virus infection. Blood 2001; 97: pp. 3171-3176
- 90. Zimmermann M., Flechsig C., La Monica N., et al: Hepatitis C virus core protein impairs in vitro priming of specific T cell responses by dendritic cells and hepatocytes. J Hepatol 2008; 48: pp. 51-60
- 91. Saito K., Ait-Goughoulte M., Truscott S.M., et al: Hepatitis C virus inhibits cell surface expression of HLA-DR, prevents dendritic cell maturation, and induces interleukin-10 production. J Virol 2008; 82: pp. 3320-3328
- 92. O’Beirne J., Mitchell J., Farzaneh F., et al: Inhibition of major histocompatibility complex class I antigen presentation by hepatitis C virus core protein in myeloid dendritic cells. Virology 2009; 389: pp. 1-7
- 93. Schulz S., Landi A., Garg R., et al: Indolamine 2,3-dioxygenase expression by monocytes and dendritic cell populations in hepatitis C patients. Clin Exp Immunol 2015; 180: pp. 484-498
- 94. Shiina M., and Rehermann B.: Cell culture-produced hepatitis C virus impairs plasmacytoid dendritic cell function. Hepatology 2008; 47: pp. 385-395
- 95. Dolganiuc A., Chang S., Kodys K., et al: Hepatitis C virus (HCV) core protein-induced, monocyte-mediated mechanisms of reduced IFN-alpha and plasmacytoid dendritic cell loss in chronic HCV infection. J Immunol 2006; 177: pp. 6758-6768
- 96. Nattermann J., Zimmermann H., Iwan A., et al: Hepatitis C virus E2 and CD81 interaction may be associated with altered trafficking of dendritic cells in chronic hepatitis C. Hepatology 2006; 44: pp. 945-954
- 97. Gondois-Rey F., Dental C., Halfon P., et al: Hepatitis C virus is a weak inducer of interferon alpha in plasmacytoid dendritic cells in comparison with influenza and human herpesvirus type-1. PLoS One 2009; 4: pp. e4319
- 98. Miyazaki M., Kanto T., Inoue M., et al: Impaired cytokine response in myeloid dendritic cells in chronic hepatitis C virus infection regardless of enhanced expression of Toll-like receptors and retinoic acid inducible gene-I. J Med Virol 2008; 80: pp. 980-988
- 99. Gerosa F., Gobbi A., Zorzi P., et al: The reciprocal interaction of NK cells with plasmacytoid or myeloid dendritic cells profoundly affects innate resistance functions. J Immunol 2005; 174: pp. 727-734
- 100. Niesen E., Schmidt J., Flecken T., et al: Suppressive effect of interleukin 10 on priming of naive hepatitis C virus-specific CD8+ T cells. J Infect Dis 2015; 211: pp. 821-826
- 101. Florimond A., Chouteau P., Bruscella P., et al: Human hepatic stellate cells are not permissive for hepatitis C virus entry and replication. Gut 2015; 64: pp. 957-965
- 102. Wang Y., Li J., Wang X., et al: Induction of interferon-lambda contributes to Toll-like receptor-3-activated hepatic stellate cell-mediated hepatitis C virus inhibition in hepatocytes. J Viral Hepat 2013; 20: pp. 385-394
- 103. Giugliano S., Kriss M., Golden-Mason L., et al: Hepatitis C virus infection induces autocrine interferon signaling by human liver endothelial cells and release of exosomes, which inhibits viral replication. Gastroenterology 2015; 148: pp. 392-402.e13
- 104. Knolle P.A., Kremp S., Hohler T., et al: Viral and host factors in the prediction of response to interferon-alpha therapy in chronic hepatitis C after long-term follow-up. J Viral Hepat 1998; 5: pp. 399-406
- 105. Ramos H.J., Lanteri M.C., Blahnik G., et al: IL-1beta signaling promotes CNS-intrinsic immune control of West Nile virus infection. PLoS Pathog 2012; 8: pp. e1003039
- 106. Negash A.A., Ramos H.J., Crochet N., et al: IL-1β production through the NLRP3 inflammasome by hepatic macrophages links hepatitis C virus infection with liver inflammation and disease. PLoS Pathog 2013; 9: pp. e1003330
- 107. Mengshol J.A., Golden-Mason L., Arikawa T., et al: A crucial role for Kupffer cell-derived galectin-9 in regulation of T cell immunity in hepatitis C infection. PLoS One 2010; 5: pp. e9504
- 108. Lunemann S., Schlaphoff V., Cornberg M., et al: NK cells in hepatitis C: role in disease susceptibility and therapy. Dig Dis 2012; 30: pp. 48-54
- 109. Khakoo S.I., Thio C.L., Martin M.P., et al: HLA and NK cell inhibitory receptor genes in resolving hepatitis C virus infection. Science 2004; 305: pp. 872-874
- 110. Romero V., Azocar J., Zuniga J., et al: Interaction of NK inhibitory receptor genes with HLA-C and MHC class II alleles in hepatitis C virus infection outcome. Mol Immunol 2008; 45: pp. 2429-2436
- 111. Golden-Mason L., Cox A.L., Randall J.A., et al: Increased natural killer cell cytotoxicity and NKp30 expression protects against hepatitis C virus infection in high-risk individuals and inhibits replication in vitro. Hepatology 2010; 52: pp. 1581-1589
- 112. Amadei B., Urbani S., Cazaly A., et al: Activation of natural killer cells during acute infection with hepatitis C virus. Gastroenterology 2010; 138: pp. 1536-1545
- 113. Pelletier S., Drouin C., Bedard N., et al: Increased degranulation of natural killer cells during acute HCV correlates with the magnitude of virus-specific T cell responses. J Hepatol 2010; 53: pp. 805-816
- 114. Kokordelis P., Kramer B., Korner C., et al: An effective interferon-gamma-mediated inhibition of hepatitis C virus replication by natural killer cells is associated with spontaneous clearance of acute hepatitis C in human immunodeficiency virus-positive patients. Hepatology 2014; 59: pp. 814-827
- 115. Corado J., Toro F., Rivera H., et al: Impairment of natural killer (NK) cytotoxic activity in hepatitis C virus (HCV) infection. Clin Exp Immunol 1997; 109: pp. 451-457
- 116. Kaser A., Enrich B., Ludwiczek O., et al: Interferon-alpha (IFN-alpha) enhances cytotoxicity in healthy volunteers and chronic hepatitis C infection mainly by the perforin pathway. Clin Exp Immunol 1999; 118: pp. 71-77
- 117. Morishima C., Paschal D.M., Wang C.C., et al: Decreased NK cell frequency in chronic hepatitis C does not affect ex vivo cytolytic killing. Hepatology 2006; 43: pp. 573-580
- 118. De Maria A., Fogli M., Mazza S., et al: Increased natural cytotoxicity receptor expression and relevant IL-10 production in NK cells from chronically infected viremic HCV patients. Eur J Immunol 2007; 37: pp. 445-455
- 119. Golden-Mason L., Madrigal-Estebas L., McGrath E., et al: Altered natural killer cell subset distributions in resolved and persistent hepatitis C virus infection following single source exposure. Gut 2008; 57: pp. 1121-1128
- 120. Yoon J.C., Shiina M., Ahlenstiel G., et al: Natural killer cell function is intact after direct exposure to infectious hepatitis C virions. Hepatology 2009; 49: pp. 12-21
- 121. Jinushi M., Takehara T., Tatsumi T., et al: Negative regulation of NK cell activities by inhibitory receptor CD94/NKG2A leads to altered NK cell-induced modulation of dendritic cell functions in chronic hepatitis C virus infection. J Immunol 2004; 173: pp. 6072-6081
- 122. Tseng C.T., and Klimpel G.R.: Binding of the hepatitis C virus envelope protein E2 to CD81 inhibits natural killer cell functions. J Exp Med 2002; 195: pp. 43-49
- 123. Crotta S., Stilla A., Wack A., et al: Inhibition of natural killer cells through engagement of CD81 by the major hepatitis C virus envelope protein. J Exp Med 2002; 195: pp. 35-41
- 124. Nattermann J., Nischalke H.D., Hofmeister V., et al: The HLA-A2 restricted T cell epitope HCV core 35-44 stabilizes HLA-E expression and inhibits cytolysis mediated by natural killer cells. Am J Pathol 2005; 166: pp. 443-453
- 125. Sene D., Levasseur F., Abel M., et al: Hepatitis C virus (HCV) evades NKG2D-dependent NK cell responses through NS5A-mediated imbalance of inflammatory cytokines. PLoS Pathog 2010; 6: pp. e1001184
- 126. Wen C., He X., Ma H., et al: Hepatitis C virus infection downregulates the ligands of the activating receptor NKG2D. Cell Mol Immunol 2008; 5: pp. 475-478
- 127. Nattermann J., Feldmann G., Ahlenstiel G., et al: Surface expression and cytolytic function of natural killer cell receptors is altered in chronic hepatitis C. Gut 2006; 55: pp. 869-877
- 128. Oliviero B., Varchetta S., Paudice E., et al: Natural killer cell functional dichotomy in chronic hepatitis B and chronic hepatitis C virus infections. Gastroenterology 2009; 137: pp. 1151-1160
- 129. Bonorino P., Ramzan M., Camous X., et al: Fine characterization of intrahepatic NK cells expressing natural killer receptors in chronic hepatitis B and C. J Hepatol 2009; 51: pp. 458-467
- 130. Ahlenstiel G., Titerence R.H., Koh C., et al: Natural killer cells are polarized toward cytotoxicity in chronic hepatitis C in an interferon-alfa-dependent manner. Gastroenterology 2010; 138: pp. 325-335.e1-2
- 131. Muhanna N., Abu Tair L., Doron S., et al: Amelioration of hepatic fibrosis by NK cell activation. Gut 2011; 60: pp. 90-98
- 132. Cheng Y.Q., Ren J.P., Zhao J., et al: MicroRNA-155 regulates interferon-gamma production in natural killer cells via Tim-3 signalling in chronic hepatitis C virus infection. Immunology 2015; 145: pp. 485-497
- 133. Golden-Mason L., Bambha K.M., Cheng L., et al: Natural killer inhibitory receptor expression associated with treatment failure and interleukin-28B genotype in patients with chronic hepatitis C. Hepatology 2011; 54: pp. 1559-1569
- 134. Ahlenstiel G., Edlich B., Hogdal L.J., et al: Early changes in natural killer cell function indicate virologic response to interferon therapy for hepatitis C. Gastroenterology 2011; 141: pp. 1231-1239
- 135. Edlich B., Ahlenstiel G., Zabaleta Azpiroz A., et al: Early changes in interferon signaling define natural killer cell response and refractoriness to interferon-based therapy of hepatitis C patients. Hepatology 2012; 55: pp. 39-48
- 136. Exley M.A., and Koziel M.J.: To be or not to be NKT: natural killer T cells in the liver. Hepatology 2004; 40: pp. 1033-1040
- 137. Deignan T., Curry M.P., Doherty D.G., et al: Decrease in hepatic CD56(+) T cells and V alpha 24(+) natural killer T cells in chronic hepatitis C viral infection. J Hepatol 2002; 37: pp. 101-108
- 138. Lucas M., Gadola S., Meier U., et al: Frequency and phenotype of circulating Valpha24/Vbeta11 double-positive natural killer T cells during hepatitis C virus infection. J Virol 2003; 77: pp. 2251-2257
- 139. Yamagiwa S., Matsuda Y., Ichida T., et al: Sustained response to interferon-alpha plus ribavirin therapy for chronic hepatitis C is closely associated with increased dynamism of intrahepatic natural killer and natural killer T cells. Hepatol Res 2008; 38: pp. 664-672
- 140. Inoue M., Kanto T., Miyatake H., et al: Enhanced ability of peripheral invariant natural killer T cells to produce IL-13 in chronic hepatitis C virus infection. J Hepatol 2006; 45: pp. 190-196
- 141. Golden-Mason L., Castelblanco N., O’Farrelly C., et al: Phenotypic and functional changes of cytotoxic CD56pos natural T cells determine outcome of acute hepatitis C virus infection. J Virol 2007; 81: pp. 9292-9298
- 142. Wahl C., Bochtler P., Schirmbeck R., et al: Type I IFN-producing CD4 Valpha14i NKT cells facilitate priming of IL-10-producing CD8 T cells by hepatocytes. J Immunol 2007; 178: pp. 2083-2093
- 143. Guidotti L.G., and Chisari F.V.: To kill or to cure: options in host defense against viral infection. Curr Opin Immunol 1996; 8: pp. 478-483
- 144. Diepolder H.M., Zachoval R., Hoffmann R.M., et al: Possible mechanism involving T-lymphocyte response to non-structural protein 3 in viral clearance in acute hepatitis C virus infection. Lancet 1995; 346: pp. 1006-1007
- 145. Missale G., Bertoni R., Lamonaca V., et al: Different clinical behaviors of acute hepatitis C virus infection are associated with different vigor of the anti-viral cell-mediated immune response. J Clin Invest 1996; 98: pp. 706-714
- 146. Gerlach J.T., Diepolder H.M., Jung M.C., et al: Recurrence of hepatitis C virus after loss of virus-specific CD4(+) T-cell response in acute hepatitis C. Gastroenterology 1999; 117: pp. 933-941
- 147. Ray S.C., Wang Y.M., Laeyendecker O., et al: Acute hepatitis C virus structural gene sequences as predictors of persistent viremia: hypervariable region 1 as a decoy. J Virol 1999; 73: pp. 2938-2946
- 148. Gruner N.H., Gerlach T.J., Jung M.C., et al: Association of hepatitis C virus-specific CD8+ T cells with viral clearance in acute hepatitis C. J Infect Dis 2000; 181: pp. 1528-1536
- 149. Lechner F., Gruener N.H., Urbani S., et al: CD8+ T lymphocyte responses are induced during acute hepatitis C virus infection but are not sustained. Eur J Immunol 2000; 30: pp. 2479-2487
- 150. Thimme R., Oldach D., Chang K.M., et al: Determinants of viral clearance and persistence during acute hepatitis C virus infection. J Exp Med 2001; 194: pp. 1395-1406
- 151. Chang K.M.: Immunopathogenesis of hepatitis C virus infection. Clin Liver Dis 2003; 7: pp. 89-105
- 152. Cooper S., Erickson A.L., Adams E.J., et al: Analysis of a successful immune response against hepatitis C virus. Immunity 1999; 10: pp. 439-449
- 153. Thimme R., Bukh J., Spangenberg H.C., et al: Viral and immunological determinants of hepatitis C virus clearance, persistence, and disease. Proc Natl Acad Sci U S A 2002; 99: pp. 15661-15668
- 154. Zubkova I., Duan H., Wells F., et al: Hepatitis C virus clearance correlates with HLA-DR expression on proliferating CD8+ T cells in immune-primed chimpanzees. Hepatology 2014; 59: pp. 803-813
- 155. Grakoui A., Shoukry N.H., Woollard D.J., et al: HCV persistence and immune evasion in the absence of memory T cell help. Science 2003; 302: pp. 659-662
- 156. Shoukry N.H., Grakoui A., Houghton M., et al: Memory CD8+ T cells are required for protection from persistent hepatitis C virus infection. J Exp Med 2003; 197: pp. 1645-1655
- 157. Thomson M., Nascimbeni M., Havert M.B., et al: The clearance of hepatitis C virus infection in chimpanzees may not necessarily correlate with the appearance of acquired immunity. J Virol 2003; 77: pp. 862-870
- 158. Kaplan D.E., Sugimoto K., Newton K., et al: Discordant role of CD4 T-cell response relative to neutralizing antibody and CD8 T-cell responses in acute hepatitis C. Gastroenterology 2007; 132: pp. 654-666
- 159. Folgori A., Spada E., Pezzanera M., et al: Early impairment of hepatitis C virus specific T cell proliferation during acute infection leads to failure of viral clearance. Gut 2006; 55: pp. 1012-1019
- 160. Lechner F., Wong D.K., Dunbar P.R., et al: Analysis of successful immune responses in persons infected with hepatitis C virus. J Exp Med 2000; 191: pp. 1499-1512
- 161. Schulze zur Wiesch J., Lauer G.M., Day C.L., et al: Broad repertoire of the CD4+ Th cell response in spontaneously controlled hepatitis C virus infection includes dominant and highly promiscuous epitopes. J Immunol 2005; 175: pp. 3603-3613
- 162. Smyk-Pearson S., Tester I.A., Klarquist J., et al: Spontaneous recovery in acute human hepatitis C virus infection: functional T-cell thresholds and relative importance of CD4 help. J Virol 2008; 82: pp. 1827-1837
- 163. Puig M., Mihalik K., Tilton J.C., et al: CD4+ immune escape and subsequent T-cell failure following chimpanzee immunization against hepatitis C virus. Hepatology 2006; 44: pp. 736-745
- 164. Mondelli M., Alberti A., Tremolada F., et al: In-vitro cell-mediated cytotoxicity for autologous liver cells in chronic non-A, non-B hepatitis. Clin Exp Immunol 1986; 63: pp. 147-155
- 165. Kato T., Esumi M., Yamashita S., et al: Interferon-inducible gene expression in chimpanzee liver infected with hepatitis C virus. Virology 1992; 190: pp. 856-860
- 166. Hoffmann R.M., Diepolder H.M., Zachoval R., et al: Mapping of immunodominant CD4+ T lymphocyte epitopes of hepatitis C virus antigens and their relevance during the course of chronic infection. Hepatology 1995; 21: pp. 632-638
- 167. Chang K.M., Thimme R., Melpolder J.J., et al: Differential CD4(+) and CD8(+) T-cell responsiveness in hepatitis C virus infection. Hepatology 2001; 33: pp. 267-276
- 168. Chang K.M., Rehermann B., McHutchison J.G., et al: Immunological significance of cytotoxic T lymphocyte epitope variants in patients chronically infected by the hepatitis C virus. J Clin Invest 1997; 100: pp. 2376-2385
- 169. Kamal S.M., Graham C.S., He Q., et al: Kinetics of intrahepatic hepatitis C virus (HCV)-specific CD4+ T cell responses in HCV and Schistosoma mansoni coinfection: relation to progression of liver fibrosis. J Infect Dis 2004; 189: pp. 1140-1150
- 170. Graham C.S., Curry M., He Q., et al: Comparison of HCV-specific intrahepatic CD4+ T cells in HIV/HCV versus HCV. Hepatology 2004; 40: pp. 125-132
- 171. Weston S.J., Leistikow R.L., Reddy K.R., et al: Reconstitution of hepatitis C virus-specific T-cellmediated immunity after liver transplantation. Hepatology 2005; 41: pp. 72-81
- 172. Berenguer M., Prieto M., Rayon J.M., et al: Natural history of clinically compensated hepatitis C virus-related graft cirrhosis after liver transplantation. Hepatology 2000; 32: pp. 852-858
- 173. McCaughan G.W., and Zekry A.: Effects of immunosuppression and organ transplantation on the natural history and immunopathogenesis of hepatitis C virus infection. Transpl Infect Dis 2000; 2: pp. 166-185
- 174. Nunez M., and Soriano V.: Hepatitis C virus (HCV) genotypes and disease progression in HIV/HCV-coinfected patients. J Infect Dis 2005; 191: pp. 1-3
- 175. Bertolino P., Trescol-Biemont M.C., Thomas J., et al: Death by neglect as a deletional mechanism of peripheral tolerance. Int Immunol 1999; 11: pp. 1225-1238
- 176. Wuensch S.A., Pierce R.H., and Crispe I.N.: Local intrahepatic CD8+ T cell activation by a non-self-antigen results in full functional differentiation. J Immunol 2006; 177: pp. 1689-1697
- 177. Kruse N., Neumann K., Schrage A., et al: Priming of CD4(+) T cells by liver sinusoidal endothelial cells induces CD25(low) forkhead box protein 3- regulatory T cells suppressing autoimmune hepatitis. Hepatology 2009; 50: pp. 1904-1913
- 178. Bull R.A., Leung P., Gaudieri S., et al: Transmitted/founder viruses rapidly escape from CD8+ T cell responses in acute hepatitis C virus infection. J Virol 2015; 89: pp. 5478-5490
- 179. Wang Y., Menne S., Jacob J.R., et al: Role of type 1 versus type 2 immune responses in liver during the onset of chronic woodchuck hepatitis virus infection. Hepatology 2003; 37: pp. 771-780
- 180. Boettler T., Spangenberg H.C., Neumann-Haefelin C., et al: T cells with a CD4+CD25+ regulatory phenotype suppress in vitro proliferation of virus-specific CD8+ T cells during chronic hepatitis C virus infection. J Virol 2005; 79: pp. 7860-7867
- 181. Rushbrook S.M., Ward S.M., Unitt E., et al: Regulatory T cells suppress in vitro proliferation of virus-specific CD8+ T cells during persistent hepatitis C virus infection. J Virol 2005; 79: pp. 7852-7859
- 182. Sugimoto K., Stadanlick J., Ikeda F., et al: Influence of ethnicity in the outcome of hepatitis C virus infection and cellular immune response. Hepatology 2003; 37: pp. 590-599
- 183. Ulsenheimer A., Gerlach J.T., Gruener N.H., et al: Detection of functionally altered hepatitis C virus-specific CD4 T cells in acute and chronic hepatitis C. Hepatology 2003; 37: pp. 1189-1198
- 184. Wedemeyer H., He X.S., Nascimbeni M., et al: Impaired effector function of hepatitis C virus-specific CD8+ T cells in chronic hepatitis C virus infection. J Immunol 2002; 169: pp. 3447-3458
- 185. Ziegler S., Skibbe K., Walker A., et al: Impact of sequence variation in a dominant HLA-A*02-restricted epitope in hepatitis C virus on priming and cross-reactivity of CD8+ T cells. J Virol 2014; 88: pp. 11080-11090
- 186. Azocar J., Clavijo O.P., and Yunis E.J.: MHC class II genes in HCV viral clearance of hepatitis C infected Hispanic patients. Hum Immunol 2003; 64: pp. 99-102
- 187. Harris R.A., Sugimoto K., Kaplan D.E., et al: Human leukocyte antigen class II associations with hepatitis C virus clearance and virus-specific CD4 T cell response among Caucasians and African Americans. Hepatology 2008; 48: pp. 70-79
- 188. Erickson A.L., Kimura Y., Igarashi S., et al: The outcome of hepatitis C virus infection is predicted by escape mutations in epitopes targeted by cytotoxic T lymphocytes. Immunity 2001; 15: pp. 883-895
- 189. Kittlesen D.J., Chianese-Bullock K.A., Yao Z.Q., et al: Interaction between complement receptor gC1qR and hepatitis C virus core protein inhibits T-lymphocyte proliferation. J Clin Invest 2000; 106: pp. 1239-1249
- 190. Yao Z.Q., Nguyen D.T., Hiotellis A.I., et al: Hepatitis C virus core protein inhibits human T lymphocyte responses by a complement-dependent regulatory pathway. J Immunol 2001; 167: pp. 5264-5272
- 191. Li S., Floess S., Hamann A., et al: Analysis of FOXP3+ regulatory T cells that display apparent viral antigen specificity during chronic hepatitis C virus infection. PLoS Pathog 2009; 5: pp. e1000707
- 192. Itose I., Kanto T., Kakita N., et al: Enhanced ability of regulatory T cells in chronic hepatitis C patients with persistently normal alanine aminotransferase levels than those with active hepatitis. J Viral Hepat 2009; 16: pp. 844-852
- 193. Sugimoto K., Ikeda F., Stadanlick J., et al: Suppression of HCV-specific T cells without differential hierarchy demonstrated ex-vivo in persistent HCV infection. Hepatology 2003; 38: pp. 1437-1448
- 194. Cabrera R., Tu Z., Xu Y., et al: An immunomodulatory role for CD4(+)CD25(+) regulatory T lymphocytes in hepatitis C virus infection. Hepatology 2004; 40: pp. 1062-1071
- 195. Li S., Jones K.L., Woollard D.J., et al: Defining target antigens for CD25+ FOXP3 + IFN-gamma- regulatory T cells in chronic hepatitis C virus infection. Immunol Cell Biol 2007; 85: pp. 197-204
- 196. Losikoff P.T., Mishra S., Terry F., et al: HCV epitope, homologous to multiple human protein sequences, induces a regulatory T cell response in infected patients. J Hepatol 2015; 62: pp. 48-55
- 197. Hall C.H., Kassel R., Tacke R.S., et al: HCV+ hepatocytes induce human regulatory CD4+ T cells through the production of TGF-beta. PLoS One 2010; 5: pp. e12154
- 198. Lapierre P., Janelle V., Langlois M.P., et al: Expression of viral antigen by the liver leads to chronic infection through the generation of regulatory T cells. Cell Mol Gastroenterol Hepatol 2015; 1: pp. 325-341
- 199. Smyk-Pearson S., Golden-Mason L., Klarquist J., et al: Functional suppression by FoxP3+CD4+CD25(high) regulatory T cells during acute hepatitis C virus infection. J Infect Dis 2008; 197: pp. 46-57
- 200. Bolacchi F., Sinistro A., Ciaprini C., et al: Increased hepatitis C virus (HCV)-specific CD4+CD25+ regulatory T lymphocytes and reduced HCV-specific CD4+ T cell response in HCV-infected patients with normal versus abnormal alanine aminotransferase levels. Clin Exp Immunol 2006; 144: pp. 188-196
- 201. Ward S.M., Fox B.C., Brown P.J., et al: Quantification and localisation of FOXP3+ T lymphocytes and relation to hepatic inflammation during chronic HCV infection. J Hepatol 2007; 47: pp. 316-324
- 202. Sturm N., Thelu M.A., Camous X., et al: Characterization and role of intra-hepatic regulatory T cells in chronic hepatitis C pathogenesis. J Hepatol 2010; 53: pp. 25-35
- 203. Ju Y., Shang X., Liu Z., et al: The Tim-3/galectin-9 pathway involves in the homeostasis of hepatic Tregs in a mouse model of concanavalin A-induced hepatitis. Mol Immunol 2014; 58: pp. 85-91
- 204. Hara M., Kingsley C.I., Niimi M., et al: IL-10 is required for regulatory T cells to mediate tolerance to alloantigens in vivo. J Immunol 2001; 166: pp. 3789-3796
- 205. Levings M.K., Sangregorio R., Galbiati F., et al: IFN-alpha and IL-10 induce the differentiation of human type 1 T regulatory cells. J Immunol 2001; 166: pp. 5530-5539
- 206. Noel C., Florquin S., Goldman M., et al: Chronic exposure to superantigen induces regulatory CD4(+) T cells with IL-10-mediated suppressive activity. Int Immunol 2001; 13: pp. 431-439
- 207. Kingsley C.I., Karim M., Bushell A.R., et al: CD25+CD4+ regulatory T cells prevent graft rejection: CTLA-4- and IL-10-dependent immunoregulation of alloresponses. J Immunol 2002; 168: pp. 1080-1086
- 208. Witsch E.J., Peiser M., Hutloff A., et al: ICOS and CD28 reversely regulate IL-10 on re-activation of human effector T cells with mature dendritic cells. Eur J Immunol 2002; 32: pp. 2680-2686
- 209. Banz A., Peixoto A., Pontoux C., et al: A unique subpopulation of CD4+ regulatory T cells controls wasting disease, IL-10 secretion and T cell homeostasis. Eur J Immunol 2003; 33: pp. 2419-2428
- 210. Sundstedt A., O’Neill E.J., Nicolson K.S., et al: Role for IL-10 in suppression mediated by peptide-induced regulatory T cells in vivo. J Immunol 2003; 170: pp. 1240-1248
- 211. Levings M.K., Gregori S., Tresoldi E., et al: Differentiation of Tr1 cells by immature dendritic cells requires IL-10 but not CD25+CD4+ Treg cells. Blood 2004; 105: pp. 1162-1169
- 212. Vieira P.L., Christensen J.R., Minaee S., et al: IL-10-secreting regulatory T cells do not express Foxp3 but have comparable regulatory function to naturally occurring CD4+CD25+ regulatory T cells. J Immunol 2004; 172: pp. 5986-5993
- 213. Zheng S.G., Wang J.H., Gray J.D., et al: Natural and induced CD4+CD25+ cells educate CD4+CD25− cells to develop suppressive activity: the role of IL-2, TGF-beta, and IL-10. J Immunol 2004; 172: pp. 5213-5221
- 214. Amaraa R., Mareckova H., Urbanek P., et al: Production of interleukins 10 and 12 by activated peripheral blood monocytes/macrophages in patients suffering from chronic hepatitis C virus infection with respect to the response to interferon and ribavirin treatment. Immunol Lett 2002; 83: pp. 209-214
- 215. Tsai S.L., Liaw Y.F., Chen M.H., et al: Detection of type 2-like T-helper cells in hepatitis C virus infection: implications for hepatitis C virus chronicity. Hepatology 1997; 25: pp. 449-458
- 216. Kimball P., Elswick R.K., and Shiffman M.: Ethnicity and cytokine production gauge response of patients with hepatitis C to interferon-alpha therapy. J Med Virol 2001; 65: pp. 510-516
- 217. Godkin A., Jeanguet N., Thursz M., et al: Characterization of novel HLA-DR11-restricted HCV epitopes reveals both qualitative and quantitative differences in HCV-specific CD4+ T cell responses in chronically infected and non-viremic patients. Eur J Immunol 2001; 31: pp. 1438-1446
- 218. Cramp M.E., Rossol S., Chokshi S., et al: Hepatitis C virus-specific T-cell reactivity during interferon and ribavirin treatment in chronic hepatitis C. Gastroenterology 2000; 118: pp. 346-355
- 219. MacDonald A.J., Duffy M., Brady M.T., et al: CD4 T helper type 1 and regulatory T cells induced against the same epitopes on the core protein in hepatitis C virus-infected persons. J Infect Dis 2002; 185: pp. 720-727
- 220. Koziel M.J., Dudley D., Wong J.T., et al: Intrahepatic cytotoxic T lymphocytes specific for hepatitis C virus in persons with chronic hepatitis. J Immunol 1992; 149: pp. 3339-3344
- 221. Accapezzato D., Francavilla V., Paroli M., et al: Hepatic expansion of a virus-specific regulatory CD8(+) T cell population in chronic hepatitis C virus infection. J Clin Invest 2004; 113: pp. 963-972
- 222. Knapp S., Hennig B.J., Frodsham A.J., et al: Interleukin-10 promoter polymorphisms and the outcome of hepatitis C virus infection. Immunogenetics 2003; 55: pp. 362-369
- 223. Mangia A., Santoro R., Piattelli M., et al: IL-10 haplotypes as possible predictors of spontaneous clearance of HCV infection. Cytokine 2004; 25: pp. 103-109
- 224. Lio D., Caruso C., Di Stefano R., et al: IL-10 and TNF-alpha polymorphisms and the recovery from HCV infection. Hum Immunol 2003; 64: pp. 674-680
- 225. Edwards-Smith C.J., Jonsson J.R., Purdie D.M., et al: Interleukin-10 promoter polymorphism predicts initial response of chronic hepatitis C to interferon alfa. Hepatology 1999; 30: pp. 526-530
- 226. Yee L.J., Tang J., Gibson A.W., et al: Interleukin 10 polymorphisms as predictors of sustained response in antiviral therapy for chronic hepatitis C infection. Hepatology 2001; 33: pp. 708-712
- 227. Kaplan D.E., Ikeda F., Li Y., et al: Peripheral virus-specific T-cell interleukin-10 responses develop early in acute hepatitis C infection and become dominant in chronic hepatitis. J Hepatol 2008; 48: pp. 903-913
- 228. Kamal S.M., Bianchi L., Al Tawil A., et al: Specific cellular immune response and cytokine patterns in patients coinfected with hepatitis C virus and Schistosoma mansoni. J Infect Dis 2001; 184: pp. 972-982
- 229. Nelson D.R., Tu Z., Soldevila-Pico C., et al: Long-term interleukin 10 therapy in chronic hepatitis C patients has a proviral and anti-inflammatory effect. Hepatology 2003; 38: pp. 859-868
- 230. Barber D.L., Wherry E.J., Masopust D., et al: Restoring function in exhausted CD8 T cells during chronic viral infection. Nature 2006; 439: pp. 682-687
- 231. Golden-Mason L., Palmer B., Klarquist J., et al: Upregulation of PD-1 expression on circulating and intrahepatic hepatitis C virus-specific CD8+ T cells associated with reversible immune dysfunction. J Virol 2007; 81: pp. 9249-9258
- 232. Iwai Y., Terawaki S., Ikegawa M., et al: PD-1 inhibits antiviral immunity at the effector phase in the liver. J Exp Med 2003; 198: pp. 39-50
- 233. Urbani S., Amadei B., Tola D., et al: PD-1 expression in acute hepatitis C is associated with HCV-specific CD8 exhaustion. J Virol 2006; 80: pp. 11398-11403
- 234. Kasprowicz V., Schulze Zur Wiesch J., Kuntzen T., et al: High level of PD-1 expression on hepatitis C virus (HCV)-specific CD8+ and CD4+ T cells during acute HCV infection, irrespective of clinical outcome. J Virol 2008; 82: pp. 3154-3160
- 235. Raziorrouh B., Ulsenheimer A., Schraut W., et al: Inhibitory molecules that regulate expansion and restoration of HCV-specific CD4+ T cells in patients with chronic infection. Gastroenterology 2011; 141: pp. 1422-1431
- 236. Raghuraman S., Park H., Osburn W.O., et al: Spontaneous clearance of chronic hepatitis C virus infection is associated with appearance of neutralizing antibodies and reversal of T-cell exhaustion. J Infect Dis 2012; 205: pp. 763-771
- 237. Radziewicz H., Ibegbu C.C., Fernandez M.L., et al: Liver-infiltrating lymphocytes in chronic human hepatitis C virus infection display an exhausted phenotype with high levels of PD-1 and low levels of CD127 expression. J Virol 2007; 81: pp. 2545-2553
- 238. Shen T., Zheng J., Xu C., et al: PD-1 expression on peripheral CD8+ TEM/TEMRA subsets closely correlated with HCV viral load in chronic hepatitis C patients. Virol J 2010; 7: pp. 310
- 239. Nakamoto N., Kaplan D.E., Coleclough J., et al: Functional restoration of HCV-specific CD8 T cells by PD-1 blockade is defined by PD-1 expression and compartmentalization. Gastroenterology 2008; 134: pp. 1927-1937
- 240. Nakamoto N., Cho H., Shaked A., et al: Synergistic reversal of intrahepatic HCV-specific CD8 T cell exhaustion by combined PD-1/CTLA-4 blockade. PLoS Pathog 2009; 5: pp. e1000313
- 241. Bengsch B., Seigel B., Ruhl M., et al: Coexpression of PD-1, 2B4, CD160 and KLRG1 on exhausted HCV-specific CD8+ T cells is linked to antigen recognition and T cell differentiation. PLoS Pathog 2010; 6: pp. e1000947
- 242. McMahan R.H., Golden-Mason L., Nishimura M.I., et al: Tim-3 expression on PD-1+ HCV-specific human CTLs is associated with viral persistence, and its blockade restores hepatocyte-directed in vitro cytotoxicity. J Clin Invest 2010; 120: pp. 4546-4557
- 243. Schlaphoff V., Lunemann S., Suneetha P.V., et al: Dual function of the NK cell receptor 2B4 (CD244) in the regulation of HCV-specific CD8+ T cells. PLoS Pathog 2011; 7: pp. e1002045
- 244. Owusu Sekyere S., Suneetha P.V., Kraft A.R., et al: A heterogeneous hierarchy of co-regulatory receptors regulates exhaustion of HCV-specific CD8 T cells in patients with chronic hepatitis C. J Hepatol 2015; 62: pp. 31-40
- 245. Seigel B., Bengsch B., Lohmann V., et al: Factors that determine the antiviral efficacy of HCV-specific CD8(+) T cells ex vivo. Gastroenterology 2013; 144: pp. 426-436
- 246. Nitschke K., Flecken T., Schmidt J., et al: Tetramer enrichment reveals the presence of phenotypically diverse hepatitis C virus-specific CD8+ T cells in chronic infection. J Virol 2015; 89: pp. 25-34
- 247. Fuller M.J., Callendret B., Zhu B., et al: Immunotherapy of chronic hepatitis C virus infection with antibodies against programmed cell death-1 (PD-1). Proc Natl Acad Sci U S A 2013; 110: pp. 15001-15006
- 248. Gardiner D., Lalezari J., Lawitz E., et al: A randomized, double-blind, placebo-controlled assessment of BMS-936558, a fully human monoclonal antibody to programmed death-1 (PD-1), in patients with chronic hepatitis C virus infection. PLoS One 2013; 8: pp. e63818
- 249. McHutchison J.G., Kuo G., Houghton M., et al: Hepatitis C virus antibodies in acute icteric and chronic non-A, non-B hepatitis. Gastroenterology 1991; 101: pp. 1117-1119
- 250. Peters T., Mohr L., Scheiffele F., et al: Antibodies and viremia in acute post-transfusion hepatitis C: a prospective study. J Med Virol 1994; 42: pp. 420-427
- 251. Netski D.M., Mosbruger T., Depla E., et al: Humoral immune response in acute hepatitis C virus infection. Clin Infect Dis 2005; 41: pp. 667-675
- 252. Molina S., Castet V., Fournier-Wirth C., et al: The low-density lipoprotein receptor plays a role in the infection of primary human hepatocytes by hepatitis C virus. J Hepatol 2007; 46: pp. 411-419
- 253. Zeisel M.B., Fofana I., Fafi-Kremer S., et al: Hepatitis C virus entry into hepatocytes: molecular mechanisms and targets for antiviral therapies. J Hepatol 2011; 54: pp. 566-576
- 254. Pestka J.M., Zeisel M.B., Blaser E., et al: Rapid induction of virus-neutralizing antibodies and viral clearance in a single-source outbreak of hepatitis C. Proc Natl Acad Sci U S A 2007; 104: pp. 6025-6030
- 255. Osburn W.O., Snider A.E., Wells B.L., et al: Clearance of hepatitis C infection is associated with the early appearance of broad neutralizing antibody responses. Hepatology 2014; 59: pp. 2140-2151
- 256. Bradley D.W., Krawczynski K., Ebert J.W., et al: Parenterally transmitted non-A, non-B hepatitis: virus-specific antibody response patterns in hepatitis C virus-infected chimpanzees. Gastroenterology 1990; 99: pp. 1054-1060
- 257. Lanford R.E., Notvall L., Barbosa L.H., et al: Evaluation of a chimpanzee colony for antibodies to hepatitis C virus. J Med Virol 1991; 34: pp. 148-153
- 258. Beach M.J., Meeks E.L., Mimms L.T., et al: Temporal relationships of hepatitis C virus RNA and antibody responses following experimental infection of chimpanzees. J Med Virol 1992; 36: pp. 226-237
- 259. Hilfenhaus J., Krupka U., Nowak T., et al: Follow-up of hepatitis C virus infection in chimpanzees: determination of viraemia and specific humoral immune response. J Gen Virol 1992; 73: pp. 1015-1019
- 260. Major M.E., Mihalik K., Fernandez J., et al: Long-term follow-up of chimpanzees inoculated with the first infectious clone for hepatitis C virus. J Virol 1999; 73: pp. 3317-3325
- 261. Bassett S.E., Thomas D.L., Brasky K.M., et al: Viral persistence, antibody to E1 and E2, and hypervariable region 1 sequence stability in hepatitis C virus-inoculated chimpanzees. J Virol 1999; 73: pp. 1118-1126
- 262. Bassett S.E., Guerra B., Brasky K., et al: Protective immune response to hepatitis C virus in chimpanzees rechallenged following clearance of primary infection. Hepatology 2001; 33: pp. 1479-1487
- 263. Major M.E., Mihalik K., Puig M., et al: Previously infected and recovered chimpanzees exhibit rapid responses that control hepatitis C virus replication upon rechallenge. J Virol 2002; 76: pp. 6586-6595
- 264. Logvinoff C., Major M.E., Oldach D., et al: Neutralizing antibody response during acute and chronic hepatitis C virus infection. Proc Natl Acad Sci U S A 2004; 101: pp. 10149-10154
- 265. Feray C., Gigou M., Samuel D., et al: Incidence of hepatitis C in patients receiving different preparations of hepatitis B immunoglobulins after liver transplantation. Ann Intern Med 1998; 128: pp. 810-816
- 266. Hjalmarsson S., Blomberg J., Grillner L., et al: Sequence evolution and cross-reactive antibody responses to hypervariable region 1 in acute hepatitis C virus infection. J Med Virol 2001; 64: pp. 117-124
- 267. Tarr A.W., Urbanowicz R.A., Hamed M.R., et al: Hepatitis C patient-derived glycoproteins exhibit marked differences in susceptibility to serum neutralizing antibodies: genetic subtype defines antigenic but not neutralization serotype. J Virol 2011; 85: pp. 4246-4257
- 268. Bailey J.R., Wasilewski L.N., Snider A.E., et al: Naturally selected hepatitis C virus polymorphisms confer broad neutralizing antibody resistance. J Clin Invest 2015; 125: pp. 437-447
- 269. Meyer K., Banerjee A., Frey S.E., et al: A weak neutralizing antibody response to hepatitis C virus envelope glycoprotein enhances virus infection. PLoS One 2011; 6: pp. e23699
- 270. Takaki A., Wiese M., Maertens G., et al: Cellular immune responses persist and humoral responses decrease two decades after recovery from a single-source outbreak of hepatitis C. Nat Med 2000; 6: pp. 578-582
- 271. von Hahn T., Yoon J.C., Alter H., et al: Hepatitis C virus continuously escapes from neutralizing antibody and T-cell responses during chronic infection in vivo. Gastroenterology 2007; 132: pp. 667-678
- 272. Dowd K.A., Netski D.M., Wang X.H., et al: Selection pressure from neutralizing antibodies drives sequence evolution during acute infection with hepatitis C virus. Gastroenterology 2009; 136: pp. 2377-2386
- 273. Osburn W.O., Fisher B.E., Dowd K.A., et al: Spontaneous control of primary hepatitis C virus infection and immunity against persistent reinfection. Gastroenterology 2010; 138: pp. 315-324
- 274. Rosa D., Saletti G., De Gregorio E., et al: Activation of naive B lymphocytes via CD81, a pathogenetic mechanism for hepatitis C virus-associated B lymphocyte disorders. Proc Natl Acad Sci U S A 2005; 102: pp. 18544-18549
- 275. Doi H., Iyer T.K., Carpenter E., et al: Dysfunctional B-cell activation in cirrhosis resulting from hepatitis C infection associated with disappearance of CD27-positive B-cell population. Hepatology 2012; 55: pp. 709-719
- 276. Racanelli V., Frassanito M.A., Leone P., et al: Antibody production and in vitro behavior of CD27-defined B-cell subsets: persistent hepatitis C virus infection changes the rules. J Virol 2006; 80: pp. 3923-3934
- 277. Sugalski J.M., Rodriguez B., Moir S., et al: Peripheral blood B cell subset skewing is associated with altered cell cycling and intrinsic resistance to apoptosis and reflects a state of immune activation in chronic hepatitis C virus infection. J Immunol 2010; 185: pp. 3019-3027
- 278. Oliviero B., Cerino A., Varchetta S., et al: Enhanced B cell differentiation and reduced proliferative capacity in chronic hepatitis C and chronic hepatitis B virus infections. J Hepatol 2011; 55: pp. 53-60
- 279. Mizuochi T., Ito M., Takai K., et al: Peripheral blood memory B cells are resistant to apoptosis in chronic hepatitis C patients. Virus Res 2011; 155: pp. 349-351
- 280. Visentini M., Conti V., Cagliuso M., et al: Persistence of a large population of exhausted monoclonal B cells in mixed cryoglobuliemia after the eradication of hepatitis C virus infection. J Clin Immunol 2012; 32: pp. 729-735
- 281. Doi H., Tanoue S., and Kaplan D.E.: Peripheral CD27-CD21- B-cells represent an exhausted lymphocyte population in hepatitis C cirrhosis. Clin Immunol 2014; 150: pp. 184-191
- 282. Oliviero B., Mantovani S., Ludovisi S., et al: Skewed B cells in chronic hepatitis C virus infection maintain their ability to respond to virus-induced activation. J Viral Hepat 2015; 22: pp. 391-398
- 283. Yan X.B., Battaglia S., Boucreux D., et al: Mapping of the interacting domains of hepatitis C virus core protein and the double-stranded RNA-activated protein kinase PKR. Virus Res 2007; 125: pp. 79-87
- 284. Ryan E.J., Stevenson N.J., Hegarty J.E., et al: Chronic hepatitis C infection blocks the ability of dendritic cells to secrete IFN-alpha and stimulate T-cell proliferation. J Viral Hepat 2011; 18: pp. 840-851
- 285. Kaukinen P., Sillanpaa M., Nousiainen L., et al: Hepatitis C virus NS2 protease inhibits host cell antiviral response by inhibiting IKKepsilon and TBK1 functions. J Med Virol 2013; 85: pp. 71-82
- 286. Sillanpaa M., Kaukinen P., Melen K., et al: Hepatitis C virus proteins interfere with the activation of chemokine gene promoters and downregulate chemokine gene expression. J Gen Virol 2008; 89: pp. 432-443
- 287. Saito T., and Gale M.: Regulation of innate immunity against hepatitis C virus infection. Hepatol Res 2008; 38: pp. 115-122
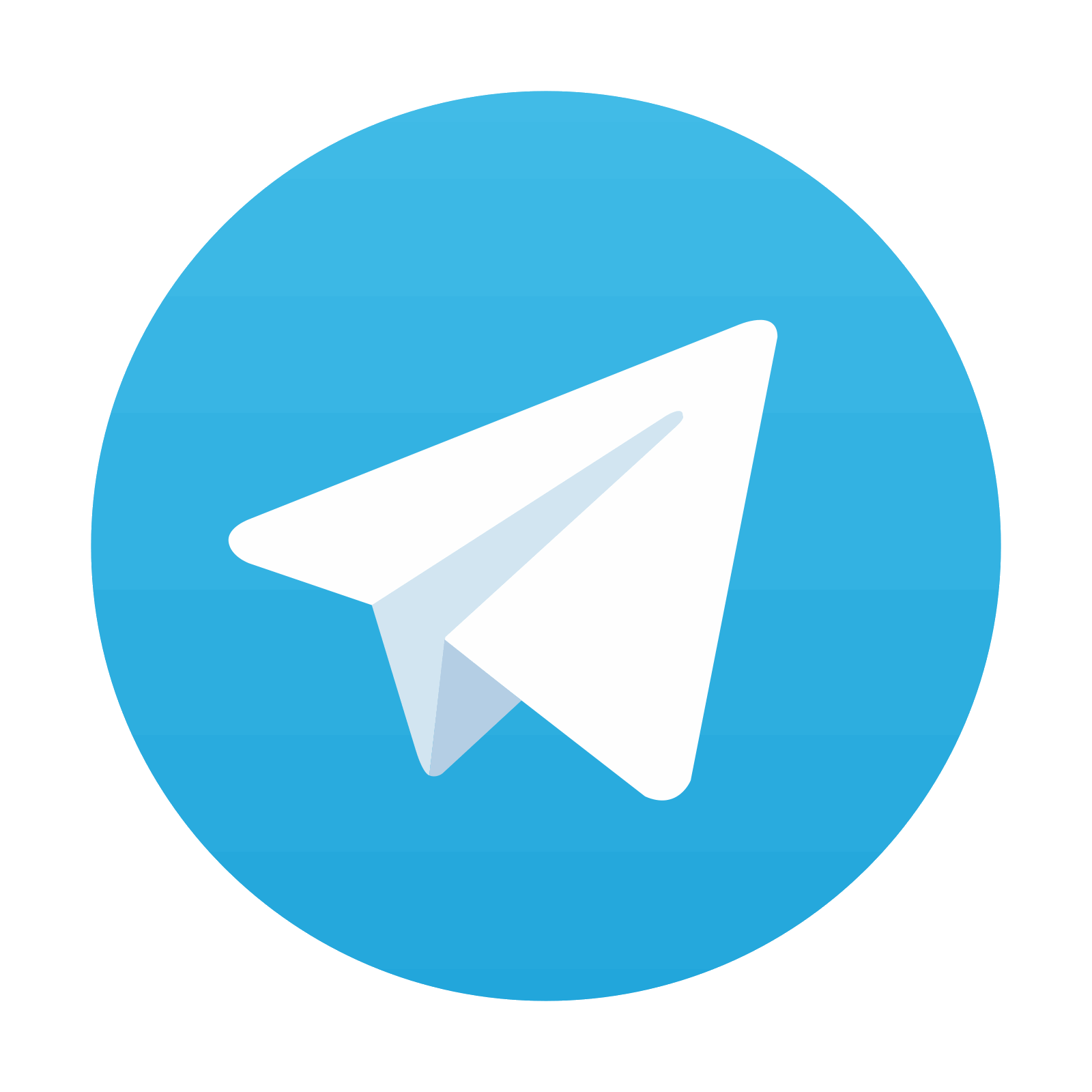
Stay updated, free articles. Join our Telegram channel
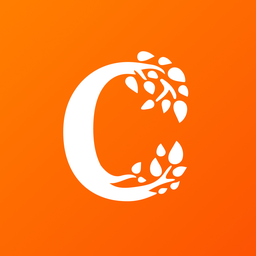
Full access? Get Clinical Tree
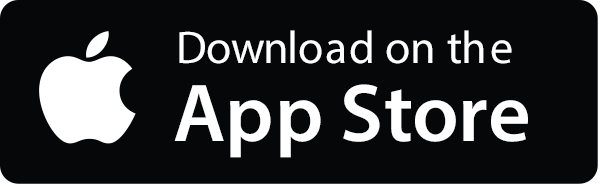
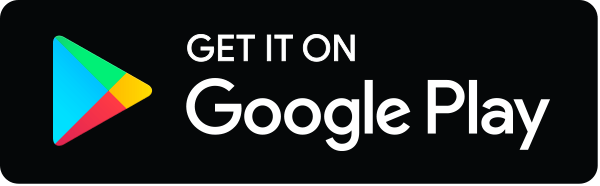