Fig. 25.1
Neo-urethra implantation and clinical outcome. (a) A cell-seeded graft sutured to the normal urethral margins from the first patient. (b) Voiding cystourethrograms of all five patients before surgery (arrows show the abnormal margins), 12 months after surgery (arrows show margins of tissue engineered urethras), and at last follow-up (arrows show margins of tissue engineered urethras)
Recognizing the unique advantages for each of the aforementioned biomaterials, studies investigating the utility of scaffolds comprised of both synthetic polymers and natural collagen-based materials, so-called hybrid scaffolds , as potential acellular or seeded grafts, are currently under evaluation in animal models [77, 78].
Tissue Engineering of Urinary Bladders
Surgical removal or replacement of the urinary bladder may be indicated for a variety of etiologies, including congenital abnormalities and subsequent end-stage bladder disease, pelvic trauma, or genitourinary malignancy. The oldest, and still today, most commonly utilized source of tissue for bladder repair or replacement are segments of bowel. However, reconstruction of the genitourinary tract with gastrointestinal tissues is associated with substantial morbidity, including excessive mucous production, development of nephrolithiasis, an increased risk of neoplasia, and the onset of metabolic abnormalities owing to the absorptive nature of gastrointestinal mucosa [79, 80].
Acellular Grafts
The earliest known surgical replacement of the bladder was reported in 1917 by Neuhoff, who used a graft of fascia for bladder augmentation in a canine model [81]. Since then, a variety of sources for tissue grafts, including skin, bladder submucosa, and small intestinal submucosa, along with multiple synthetic materials, such as plastic molds, polyvinyl sponge, tetrafluoroethylene, and collagen matrices have been evaluated for bladder replacement [82]. These substrates ultimately proved to be inadequate, as they were unable to demonstrate the mechanical, functional, and/or biocompatibility properties needed for bladder reconstruction. More recently, tissue engineering and regenerative medicine strategies have been applied towards the bladder, including the use of biomaterials derived from collagen matrices and synthetic polymers as scaffolds for regeneration.
One of the earliest reports of the use of collagen as a bladder substitution material consisted of a collagen/vicryl composite membrane to repair defects [83]. SIS was also used for augmentation cystoplasty in rat and canine models [84, 85]. The investigators reported histologic evidence of regeneration of urothelium, smooth muscle, and serosa along the implanted scaffold. Canine bladders demonstrated similar functionality to control animals on urodynamics studies. In 1997, Probst and colleagues used a rat model to perform partial cystectomy and grafting with homologous acellular bladder matrix, demonstrating neovascularization of the graft and regeneration of bladder urothelium and smooth muscle cells at 8 weeks [86].
The ability to successfully regenerate both the luminal urothelial and smooth muscle layers of the bladder on an acellular naturally-derived scaffold is somewhat variable in the literature. Investigations as recent as 2008 report successful regeneration of bladder urothelium, but incomplete or absent regeneration of the smooth muscle layer when using natural collagen-based scaffolds [87, 88]. Moreover, limitations in maximum scaffold size have been suggested, as investigators who have used both acellular and seeded SIS to replace the bladder in a canine model that has undergone subtotal cystectomy reported severe graft shrinkage, minimal regeneration of urothelium, and inflammatory infiltrate and smooth muscle hypertrophy on histological examination [89]. In a clinical investigation, five exstrophic pediatric patients presenting with poor bladder function underwent augmentation cystoplasty with an acellular SIS scaffold [90]. Although functional parameters, such as bladder capacity and compliance, increased by upwards of 30% compared to the pre-operative state, this did not translate to a clinically meaningful improvement in the duration of dry intervals reported by patients. Moreover, histological analysis of grafts following implantation revealed diminished presence of bladder smooth muscle. Due to the challenges encountered with acellular matrices, several modifications to enhance their preparation have been proposed, including incubating scaffolds in bioreactors that simulate physiological stretch of the bladder wall or exposing three-dimensional urothelial cultures to cyclical increases and rapid decreases of pressure to simulate physiological bladder filling and rapid emptying [91, 92].
Tissue Engineered Bladders
Harvesting autologous urothelium, expanding cells in culture, and then seeding those cells onto a biodegradable polymer scaffold for eventual replacement of genitourinary tissues was first described in 1992 [93]. In this proof of concept study, culture-expanded rabbit urothelial cells were seeded onto nonwoven PGA mesh and implanted into mice. This model was further expanded on by demonstrating the ability of harvested human urothelium and bladder smooth muscle cells to be expanded in vitro, seeded onto biodegradable PGA scaffolds, and implanted in vivo to create urological structures consisting of both cell types [94]. Subsequent studies in the canine model demonstrated improved regenerative capacity of allogenic acellular bladder matrix scaffolds when grafts were seeded with autologous cells prior to implantation. Specifically, investigators harvested bladder urothelium and smooth muscle cells, isolated each cell type and expanded them separately in culture, and seeded the luminal surface of the scaffolds with urothelium and the outer surface with smooth muscle [95]. Additionally, when compared to animals receiving unseeded acellular matrices, augmentation with seeded matrices resulted in a significantly greater increase in bladder capacity (99% vs. 30% for acellular matrices). Seeded matrices also retained their size compared to acellular matrices, which demonstrated graft contraction and shrinkage. In another study, culture-expanded autologous urothelial and smooth muscle cells were seeded on the luminal and exterior surfaces, respectively, of biodegradable PGA scaffolds molded into the shape of a bladder [96]. These scaffolds were then implanted into a canine model after animals underwent trigone sparing cystectomy. At 11 months following implantation, organs demonstrated filling capacities of upwards of 95% of the baseline, pre-cystectomy bladder volume, physiological properties of elasticity, and normal bladder histology consisting of organized urothelium, muscle fiber, and submucosal layers, making this the first report of a successfully tissue-engineered autologous hollow organ.
This technology was eventually translated into the clinical setting when seven patients with myelomeningocele were identified to undergo cystoplasty with tissue-engineered autologous bladders [97]. Autologous bladder urothelium and smooth muscle were harvested and individually expanded in vitro. Scaffolds fashioned from a combination of collagen and PGA were molded into the shape of a bladder; the luminal surface and outer surface were seeded with patient-derived urothelium and smooth muscle cells, respectively. Constructs used for reconstruction cystoplasty with the addition of an omental wrap resulted in increased bladder volume and compliance, along with decreased LPP at an average of 46 months post-operative follow-up (Fig. 25.2). Moreover, the cellular phenotype and structural architecture of the implanted constructs resembled that of histologically normal bladder tissues. The most frequently encountered complications of cystectomy, including mucous production, metabolic abnormalities, neoplasia, or nephrolithiasis, were absent in this cohort, demonstrating the safety and feasibility of using tissue engineering technologies for the structural and functional regeneration of human bladders. Although phase II trials, which utilized cell expansion and manufacturing scale-up methods, did not show functional differences long term, the phase I patients continue to do well and Phase III trials are currently in preparation with a modified scale-up protocol [98, 99].


Fig. 25.2
Construction of engineered bladder. Scaffold seeded with cells (a) and engineered bladder anastomosed to native bladder with running 4–0 polyglycolic sutures (b). Implant covered with fibrin glue and omentum (c)
Tissue-Engineered Urinary Conduits
Patients who undergo cystectomy and urinary diversion face exceptionally high rates of perioperative complications (50–70%), 90-day hospital readmission (25%), intensive care unit admission (20%), and perioperative mortality (5%) [100]. To this end, tissue engineered urinary conduits (TEUC) have been proposed with the goal of obviating the need for elective surgical manipulation of the gastrointestinal tract, arguably one of the most morbid aspects of this surgery. One of the earliest descriptions of TEUCs was made in 2007 by Drewa, who constructed a conduit in a rat model using SIS seeded with culture-expanded fibroblasts [101]. In another preclinical study using a porcine model, conduits were created from tubularized constructs of type I bovine collagen and a synthetic polymer mesh and subsequently seeded with porcine urothelial cells [102]. While implanted conduits demonstrated growth of luminal urothelium and neovascularization on histological analysis, the rates of animal survival and creation of a functional urostomy was only 80% and 50%, respectively. Additional work using a porcine model was performed by seeding biodegradable polymer scaffolds with autologous adipose- and peripheral blood-derived smooth muscle cells [103]. Histological analysis of tubular conduits following their implantation revealed de novo regeneration of “urinary-like neo-tissue” with similar morphologic appearance to native bladder.
TEUCs have also been evaluated in a phase I open label clinical trial in nine patients with bladder cancer undergoing radical cystectomy and urinary diversion. Biodegradable PGA polymer mesh constructs coated with a PLGA copolymer were tubularized to create a 20 cm long conduit, which was subsequently seeded with autologous adipose-derived smooth muscle cells and implanted at the time of surgery [104]. Investigators utilized the omental pedicle for vascularization of the TEUC and optimized surgical techniques for ureteral implantation and stoma creation. Histologically, the implanted conduits demonstrated regeneration of urothelium and smooth muscle along the tract. Long-term follow-up detailing the patency and structural integrity of these TEUCs, along with rates of complications, are pending.
Tissue Engineered Female Genital and Reproductive Tissues
A variety of conditions may result in either absence or loss of female genital and reproductive tissues . Congenital disorders, such as Mayer-Rokitansky-Kuster-Hauser syndrome (MRKHS) , cloacal malformations, or intersex disorders, may result in vaginal aplasia, while acquired disorders, such as malignancies or trauma, may lead to substantial structural and/or functional organ damage. Patients requiring vaginal reconstruction commonly undergo McIndoe vaginoplasty , where a pelvic canal is created from the potential space posterior to the urethra and urinary bladder and anterior to the rectum. In creating this neovagina, a tissue substitute is needed to line the wall of this cavity and aid in its functionality. Multiple sources of tissue have been previously evaluated, including skin grafts, buccal mucosa, vaginal epithelium, acellular dermal matrix, and acellular porcine SIS [105–109]. While some of these tissues have demonstrated satisfactory take to native tissue beds, they have typically only resulted in epithelial regeneration with an absence of an adequate muscle layer resulting in eventual graft stenosis or contracture and need for dilation. Reconstruction of vaginal structures has also been attempted using segments of bowel; however, similar to what has been previously reported in the setting of urinary diversions or bladder reconstruction, use of intestine to reconstruct female genital tissues has also been associated with complications, such as excessive mucous production, poor hygiene, and risk for onset of neoplasia [110, 111]. To attempt to overcome these challenges, tissue engineering strategies have been applied towards the regeneration of vaginal organs, with the objective to provide patients with both structural and functional organ replacement and an improved quality of life over currently available options.
The first report of successfully engineering vaginal tissues with cells was published in 2003. In this proof of concept study, vaginal epithelial and smooth muscle cells were harvested from female rabbits and individually expanded in culture [112]. These cells were then seeded onto biodegradable PGA scaffolds and constructs were implanted into female mice. At 4 weeks post-implantation, grafted cell seeded scaffolds demonstrated neovascularization and tissue-level organization of vaginal epithelium and smooth muscle; additionally, regenerated vaginal tissues demonstrated functional contractile properties similar to that of control tissues when subjected to electrical stimulation. In a follow up study, the feasibility of engineering a functional autologous vagina was demonstrated using a rabbit model [113]. Harvested and culture-expanded vaginal epithelial cells and smooth muscle cells were seeded onto the inner surface and outer surface, respectively, of PGA scaffolds constructed to resemble a vaginal canal. At 6 months following implantation, histological examination of constructs demonstrated neovascularization and organized epithelial and smooth muscle. Moreover, vaginal canals remained patent without the development of strictures and tissue functionality testing demonstrated appropriate physiological responses to either stimulation with electrical currents or an adrenergic agonist.
This technology was further developed with the first report of successful tissue engineered autologous vaginal organs in human patients in 2014. Four patients with vaginal aplasia secondary to MRKHS underwent vulvar tissue biopsy, followed by isolation of epithelial and smooth muscle cells and expansion in culture [114]. SIS scaffolds were molded to the unique pelvic anatomy for each patient and subsequently seeded with autologous epithelial cells on the inner surface of the vaginal canal and autologous smooth muscle cells on the outer surface. Following growth and maturation of these constructs in an incubator, tissue engineered neovaginas were surgically implanted into patients. At 8 years follow up, annual vaginal biopsies consistently demonstrated organized vaginal histology consisting of epithelial, submucosal, and smooth muscle layers. Before and after pelvic cross-sectional imaging demonstrated the severity of vaginal aplasia pre-operatively and the durability of regenerated tissues post-operatively (Fig. 25.3). Moreover, adequate functional outcomes were reported based on the Female Sexual Function Index questionnaire. Certainly, the use of seeded constructs for female genital organ regeneration is promising and necessitates further investigation.


Fig. 25.3
Preoperative and Postoperative MRI images. (a) Preoperative MRI images show absence of vaginal organs. (b) MRIs 1 year after surgery show engineered vaginal organs. (c) Latest MRI images up to 8 years after surgery (boxes within the MRIs show engineered vaginal organs)
Investigations directed at engineering uterine tissue have also been performed [115]. In a similar fashion as described above, uterine epithelial cells and smooth muscle were harvested from female rabbits, individually expanded in culture, and seeded onto biodegradable polymer scaffolds which were constructed to resemble a uterine cavity. Seeded scaffolds were implanted into corresponding autologous animals. Histologic, molecular, and biomechanical studies at 6 months following implantation demonstrated the presence of normal uterine tissue components and normal uterine functionality.
Tissue Engineering of Renal Structures
Perhaps one of the greatest medical achievements over the past half-century has been the development of surgical techniques that have made renal transplantation feasible. First reported in 1955, renal transplantation is the gold standard and only curative therapy available for end-stage renal disease [116]. However, a severe donor organ shortage for renal transplantation exists and, although substantial advancements have been made, immunosuppressive pharmacology regimens are still associated with significant morbidity and mortality. Transplant recipients are also at risk of rejection or loss of graft function over time. Currently, patients with renal failure are most commonly treated with dialysis as a renal replacement therapy , but, this too, is highly morbid. Given its highly complex architecture and cellular heterogeneity, the kidney is arguably the most difficult organ to regenerate in the genitourinary system. Efforts in renal tissue engineering have sought to create tissues or whole organs capable of regenerating diminished renal function, with the ultimate objective of providing an alternative therapy to dialysis or transplantation. Towards this goal, investigators have proposed several concepts for renal tissue regeneration, ranging from therapies derived from stem cells and embryologic precursors to total functional renal replacement with decellularized naturally-derived scaffolds.
Cell-Based Therapies
The complexities of renal stromal architecture and the heterogeneous cell types which comprise the kidney make developing cellular therapies for renal disease inherently challenging. Many investigations have revolved around mesenchymal stem cells (MSC) for tissue engineering with the objective of harnessing their capacity to differentiate into one of many different cell types as a therapeutic option. The restorative capabilities of MSCs are often studied in the setting of acute kidney injury (AKI) or chronic kidney disease (CKD) , where the number of primary renal cells remaining may be inadequate to achieve proper renal function.
Bone marrow-derived MSCs have been demonstrated to be capable of differentiating into, and ultimately regenerating, several cell lineages, including glomerular endothelial cells in the setting of significant damage [117]. Bone marrow-derived MSCs also have a role in renal development, specifically in nephron formation, when stimulated by a variety of nephrogenic signals following injection into an embryologic rat metanephros [118]. However, this report generated controversy as to whether the primary cell type responsible for kidney regeneration in the setting of AKI is intrarenal versus extrarenal. Additional experimentation using a renal ischemia-reperfusion injury mouse model demonstrated a majority of regenerated cells arose from renal tubular epithelial cells originating from the host [119].
Multiple animal models have been utilized to determine the renoprotective capabilities of systemically administered bone marrow-derived MSCs in the setting of renal injury [120, 121]. In a mouse model for renal injury induced by cisplatin administration, injected MSCs honed to the damaged kidney and differentiated into tubular epithelial cells [122]. Evidence of MSC induced restoration of renal structure and function were observed with enhanced rates of renal tubular proliferation and significant reductions in serum urea following MSC administration. In a rat model for ischemia-reperfusion AKI, early administration of fluorescently labeled MSCs following injury resulted in their localization to the basement membranes of glomeruli, as identified by in vivo two-photon laser confocal microscopy [123]. Several renoprotective effects, including recovery of renal function, high rates of proliferation, and low rates of apoptosis, were reported following MSC administration. Human cord blood MSCs have also been delivered to a mouse model of cisplatin-induced renal injury, resulting in production of pro-regenerative growth factors and inhibition of inflammatory mediators [124].
Mesenchymal stem cells as a renoprotective therapeutic have been translated into the clinic for evaluation in a variety of settings. The immunomodulatory effects of MSCs were first evaluated in a clinical trial of eight patients with steroid-resistant graft versus host disease (GVHD), resulting in resolution of disease in six patients and significantly improved survival compared to a matched cohort not receiving MSCs [125]. A subsequent multi-center, phase II clinical trial evaluating MSCs for treatment of steroid-refractory, acute GVHD treated 55 patients with culture expanded MSCs [126]. Overall, a complete response was achieved in 30 patients and nine additional patients demonstrated clinical improvement; there were no reported side effects or toxicities associated with infusion of MSCs. In a randomized, controlled clinical trial, living-related kidney transplant recipients who received pre-transplant induction therapy with autologous MSCs had a significantly lower rate of acute graft rejection, a significantly higher rate of recovery of renal function (eGFR) at 1 month post-operatively, and a significantly lower rate of opportunistic infection within the first year post-operatively compared to patients who underwent induction therapy with standard anti-IL2 receptor antibodies [127].
Other investigations have sought to isolate renal cell types of certain functionality as a targeted therapeutic towards a specific aspect of renal dysfunction. Whereas currently available therapies for anemia secondary to end-stage renal disease (ESRD) necessitate regular administration of recombinant erythropoietin (Epo), cell-derived therapies could potentially be used to treat anemia in this setting. Investigators first isolated and expanded in culture renal cells from mice that stably expressed Epo and later demonstrated these cells were capable of regulating expression of Epo in response to their environmental oxygen tension [128, 129]. This concept was further developed using a model for chronic kidney injury and human primary kidney cells enriched with renal cells expressing Epo [130]. These cells were introduced following injury, resulting in significant improvement of renal function and reduction of renal injury markers, such as urinary albumin, urinary kidney injury molecule-1 (a tubular injury marker), and 8-hydroxy-deoxyguianosine (an oxidative DNA marker). As a mechanism for delivery of cell-based therapies, a three-dimensional collagen-based culture system was developed to enable in vitro generation of renal structures comprised of primary renal cells [131]. Glomeruli and renal tubules, identified by positive Tamm-Horsfall protein staining, developed as early as 1 week in culture. Further studies involved expanding isolated human primary renal cells in culture and constructing in vitro three-dimensional renal cell cultures [132]. These functional three-dimensional cultures were subsequently implanted in a rat kidney model, surviving in vivo for up to 6-weeks post-implantation.
Transplantation of Metanephroi
Another potential mechanism for regeneration of renal function is through transplantation of embryological precursors, such as the metanephros. Metanephroi were first transplanted into cortical tunnels in a mouse model by Woolf et al. in 1990 and subsequent investigations demonstrated development of new nephrons, neovascularization, and glomerular and tubular cytodifferentiation when metanephroi were implanted subcapsularly in a mature rat host [133, 134]. In another investigation, embryologic day 15 (E15) metanephroi were transplanted either subcapsularly or into the omentum of adult rats, demonstrating renal differentiation, cortical and meduallary architecture, neovascularization, and production of urine [135]. The same group later reported implanted metanephroi into rat omentum are viable for upwards of 32 weeks [136]. Further studies transplanted E15 metanephroi from rats into an adult rat omentum, with investigators performing unilateral nephrectomy at the time of the initial implantation and contralateral nephrectomy 20 weeks later to create a model of end-stage renal disease [137]. Animals that received transplanted metanephroi and underwent ureteroureterostomy to create a continuous urinary tract demonstrated significantly prolonged survival. This was the first report to illustrate transplanted metanephroi were capable of prolonging survival.
Eventually, human embryologic metanephroi were utilized as a renal precursor and transplanted into kidneys of a mouse model [138]. These implants differentiated into functional nephrons that produced dilute urine, but did not generate ureters. Further work in this area involved in vitro culturing of isolated metanephric mesenchyme and ureteric bud tissues derived from rat metanephroi [139]. When cultured individually, both mesenchymal- and ureteral-derived tissues were successfully propagated and grew to the approximate size of their progenitors; moreover, combining propagated ureteric buds with fresh-harvested mesenchymal tissues in vitro generated a contiguous neokidney with identical morphology to the whole rat kidney rudiment. This same group later developed a stepwise technique for inducing budding of an epithelial tubule and then combining it with mesenchymal tissues in vitro [140]. When implanted into an adult rat model, the recombined tissue demonstrated evidence of early neovascularization and development of glomeruli. These studies have proven the feasibility of developing a metanephros-like structure by culturing ureteral buds and mesenchymal tissues, indicating the potential for propagation of the metanephros under these conditions as a technique for engineering renal tissues for substitution.
As techniques for developing and culturing renal precursors advanced, investigators sought to further elucidate the functional benefits which could potentially be acquired through transplantation of metanephroi. In 2012, a group from Japan published multiple reports describing the effect of metanephroi transplantation in a rat models. Specifically, transplanted metanephroi expressed increased levels of renin, increased plasma renin activity, and maintained mean arterial blood pressures in a rat hypotension model [141]; expressed increased levels of Epo in a rat anemia model [142]; and prevented progression of vascular calcification in a rat chronic renal failure model [143]. Cumulatively, these experiments indicate the potential for transplanted renal precursors to not only promote neovascurization and nephrogenesis in renal tissues, but to also regenerate lost renal function in a diseased kidney.
In Situ Development of Renal Units
In vitro renal units have also been proposed as a potential renal replacement therapy. These scaffolds could ideally be seeded with autologous cells to prevent complications associated with immune system rejection or immunosuppression seen in transplant recipients. The realization of tissue engineered renal units was not only dependent on the development of techniques for growth and expansion of renal cells in culture, but also the development of biomaterials scaffolds to serve as adequate vehicles for the eventual delivery of these regenerative therapies. Early advancements in this area were made by successfully culturing individual populations of renal cells harvested from rabbits, including proximal tubules, glomeruli, and distal tubules [144]. Cells were expanded in culture, seeded both individually and as mixed cultures on biodegradable PGA scaffolds , and subsequently implanted into a mouse model. Seeded cells were able to successfully attach to the polymer scaffold and histologic examination demonstrated progressive organization of nephrons. However, it could not be concluded whether the observed tubular structures within polymer fibers regenerated de novo from previously dissociated renal elements or whether they were remnants of intact tubular structures that survived harvesting and expansion in culture. To this end, renal cells were harvested from mice, culture-expanded, and individually isolated cells were seeded on biodegradable polymer scaffolds for implantation into immune-competent syngenic hosts [145]. Histological analyses of the implants demonstrated renal epithelial cells developed into tubules over time by first generating a solid, cord-like structure and then canalizing to create a hollowed core. Further examination of cell types confirmed the ability of these individually isolated cells to reconstitute renal tubular structures comprised of proximal tubules, distal tubules, loop of Henle, collecting tubules, and collecting ducts.
In another investigation, a tubular polycarbonate device was used as a scaffold for renal cells which had been harvested from mice and culture-expanded [146]. The device was subcutaneously implanted in a mouse model, while the other end was fed into a Silastic catheter that entered into a reservoir. When the device was evaluated histologically, well-organized glomeruli and tubular structures, including proximal and distal tubular cells and loop of Henle, were observed in the setting of widespread neovascularization. Osteopontin and fibronectin were identified on immunohistochemistry staining of tubular cells and regions of extracellular matrix, respectively. Analysis of fluid collected from the reservoir revealed significantly elevated mean concentrations of uric acid (66 mg/dL, vs. 2 mg/dL in serum) and creatinine (27.91 mg/dL, vs. 4.49 mg/dL in serum) compared to those found in serum. Overall, results from this investigation proved single renal cells are able to form complex, multicellular structures and functional renal units, which demonstrate unidirectional secretion of solutes and concentration of uric acid in the form of a urine-like fluid.
An extracorporeal renal tubular assist device (RAD) has also been developed which functions in a complementary fashion to hemodialysis units. The RAD is comprised of a multi-fiber bioreactor in which a confluent monolayer of proximal tubule cells are seeded. In the setting of hemodialysis, blood is first processed through a conventional hemofilter and is then directed towards the RAD for filtration across the fibers of the device as a means to provide supplemental cellular metabolic functionality to hemofiltration. Using porcine renal tubular cells in a canine model in which acute uremia was induced, the RAD resulted in increases in both ammonia excretion and plasma 1,25-dihydroxyvitamin D3 [147]. A RAD comprised of monolayers of human-derived proximal tubule cells was evaluated clinically in a phase I/II trial in ten patients admitted to the ICU with AKI [148]. Although these patient’s likelihood of pre-treatment survival was low secondary to comorbidities and multiple organ failures in an intensive care setting, 60% of the patients who used the RAD survived past 30 days. A follow-up randomized, controlled phase III clinical trial was performed comparing RAD with hemofiltration (n = 40) versus hemofiltration alone (n = 18) for patients admitted to the ICU with AKI [149]. Those who were randomized to treatment with the RAD and hemofiltration had significantly improved survival at 180 days and demonstrated earlier recovery of renal function, although this outcome was non-significant.
Renal Tissue Regeneration Through Therapeutic Cloning
While prior strategies for renal tissue regeneration have relied on stem cells, embryologic precursors, or allogenic renal cells as a biologic starting material, somatic cell nuclear transfer has also been evaluated as a potential cell source for regenerative therapies. Nuclear material was extracted from harvested bovine fibroblasts and subsequently transferred into unfertilized bovine oocytes that had undergone enucleation [150]. From these cloned embryos, renal cells were isolated, culture-expanded, and seeded onto three-dimensional biodegradable scaffolds, which were then implanted into the exact animal from which the original cells were harvested. Twelve weeks following implantation, these functioning renal units were capable of secreting solutes unidirectionally, concentrating urea nitrogen and creatinine, and producing urine (Fig. 25.4). Additionally, glomerular architecture and tubular structures were identified on histological examination and the tissues regenerated in this setting were genetically identical to the animal from which the cells were originally harvested. Results from this investigation were the first to demonstrate therapeutic cloning techniques can produce viable cells for in vitro expansion and subsequent seeding onto biodegradable scaffolds for implantation and regeneration of tissues in vivo.


Fig. 25.4
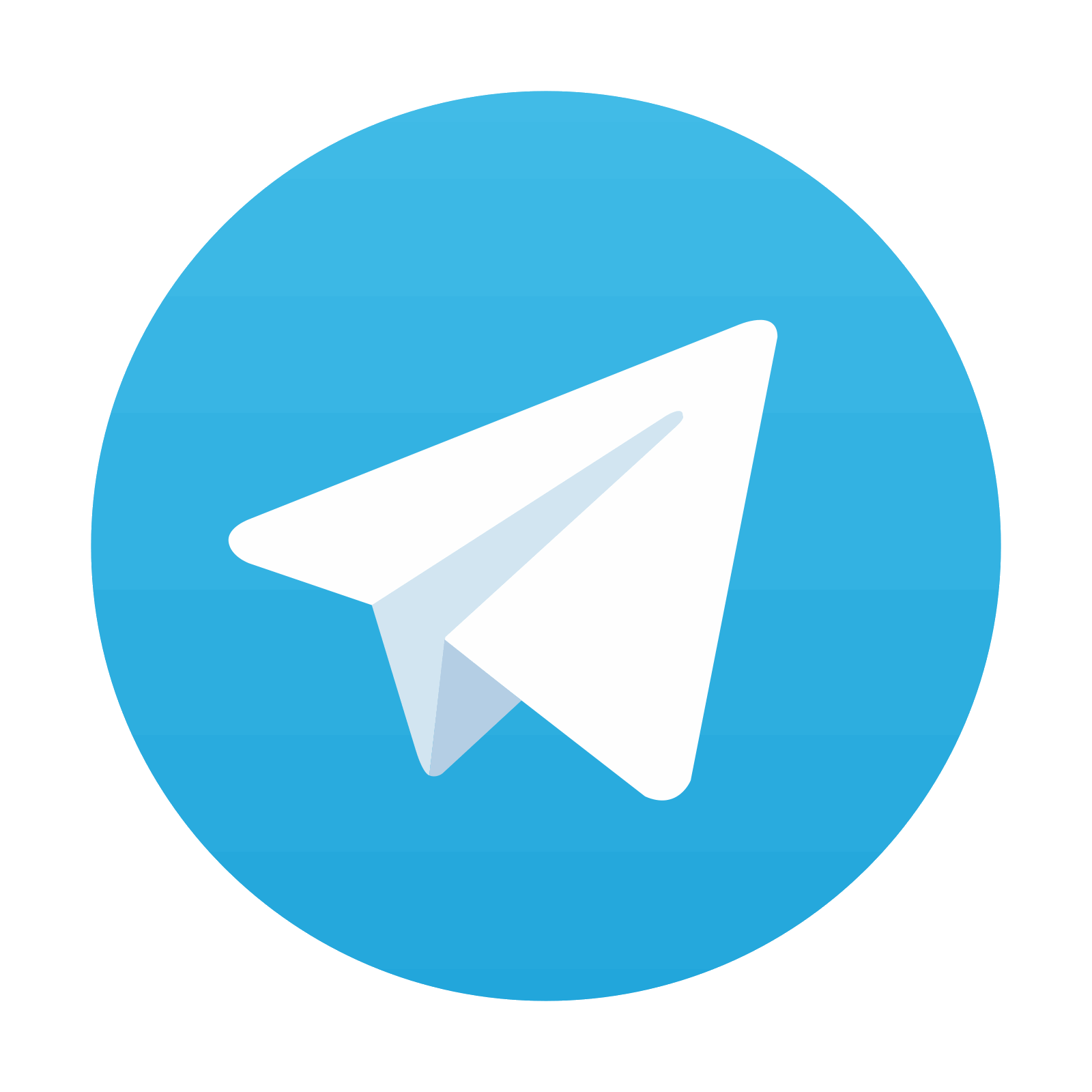
Illustration of tissue engineered renal unit (top left); Unit seeded with cloned cells and retrieved 3 months after implantation demonstrating accumulation of urine-like fluid (top left); Renal explants demonstrated a clear unidirectional continuity between the mature glomeruli, their tubules, and the polycarbonate membrane (bottom left); and ELISpot analyses of the frequencies of T cells that secrete IFN-gamma after primary and secondary stimulation with allogenic renal cells, cloned renal cells, or nuclear donor fibroblasts (bottom right)
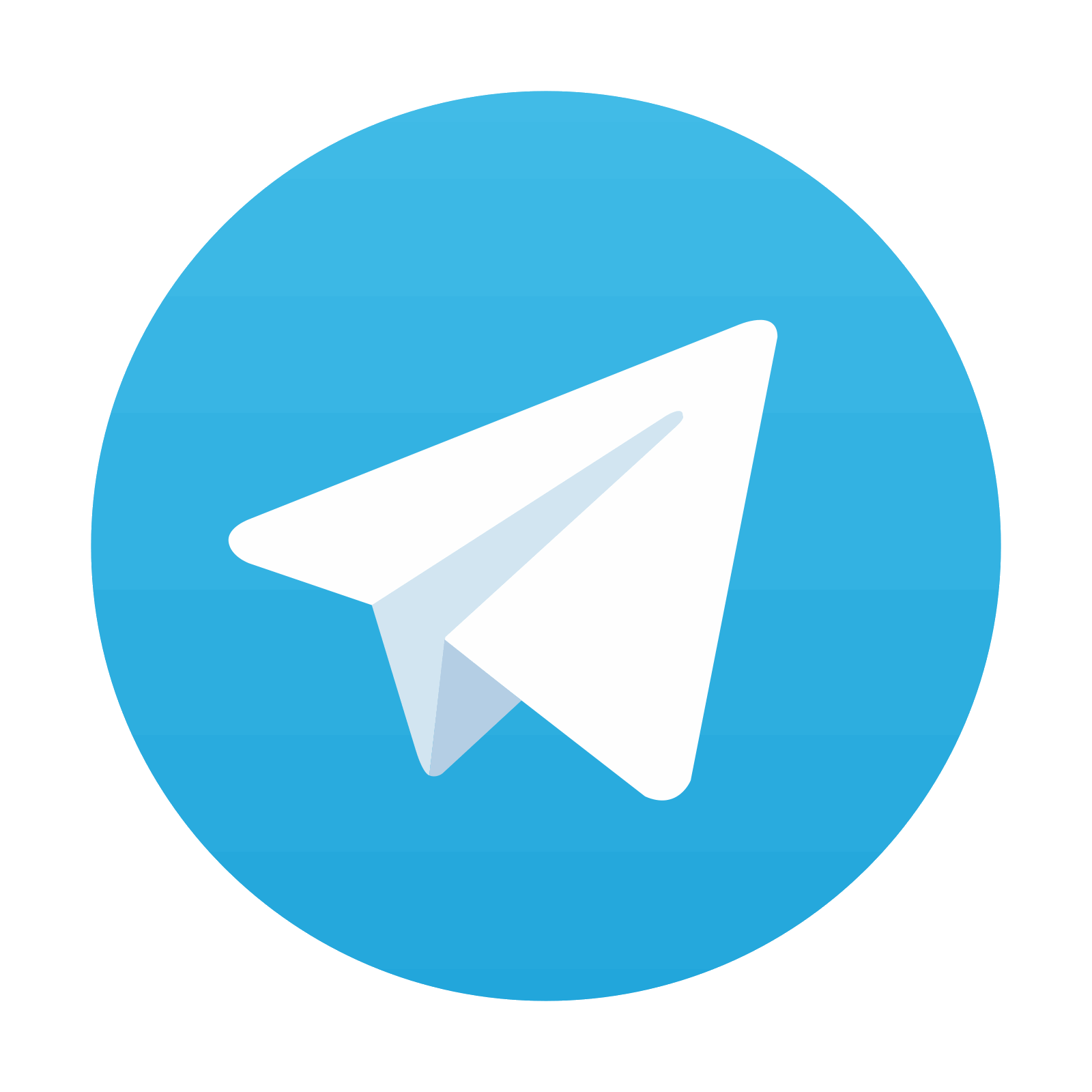
Stay updated, free articles. Join our Telegram channel
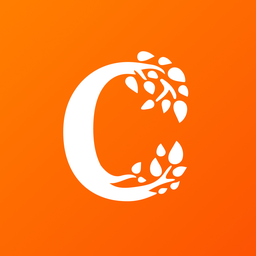
Full access? Get Clinical Tree
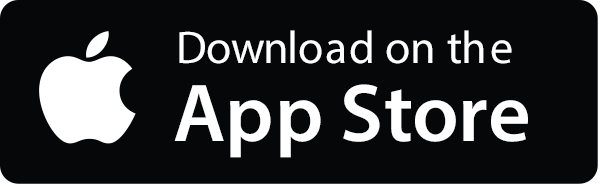
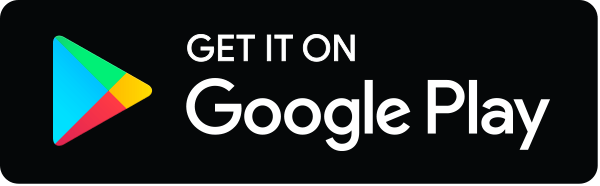
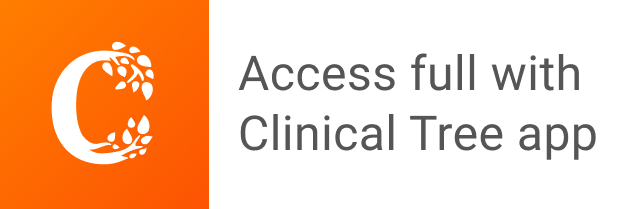