Historical Background
The study of histocompatibility accelerated during the 1960s when the pioneers of clinical kidney transplantation recognized that graft destruction was mediated through immunologic mechanisms. In 1961 the introduction of chemical immunosuppression, first 6-mercaptopurine followed soon after by azathioprine and steroids, enabled short and medium-term success. However, 40% to 50% of deceased donor transplants were lost because of immediate or early graft failure due to irreversible rejection in the first year and thereafter there was an insidious decline in graft function. These early experiences severely limited the success of human allotransplantation and led to the study of compatibility of transplanted tissue, which, over the next 40 years gave rise to the specialty of “histocompatibility and immunogenetics” (H&I).
The first human leukocyte antigens (HLA) were discovered in 1958 and subsequent years by Jean Dausset, Rose Payne, and Jon van Rood. Later, many more HLA antigens were characterized using antibodies in sera obtained from multiparous women and from patients after multiple blood transfusions. Such antibodies were also demonstrated in recipient sera after transplant rejection, and antibodies reactive with donor lymphocytes present before renal transplantation, either detected by leukoagglutination or by cytotoxicity, were found to be associated with hyperacute rejection (HAR). HLA was quickly recognized as the human equivalent of the major histocompatibility complex (MHC), previously identified in inbred rodents, the products of which control the recognition of self and foreign antigens.
The HLA System
The HLA system encoded on the short arm of chromosome 6 is the most intensively studied region of the human genome. The region spans over four megabases and contains in excess of 250 expressed genes, making it the most gene-dense region characterized to date. Approximately 28% of these genes encode proteins with immune-related functions, making the region of particular relevance to transplant clinicians and immunologists.
HLA has a central role in immune recognition for defense against foreign pathogens and neoplasia, mediating T cell signaling through the presentation of self and foreign antigens in the form of short protein fragments (peptides) recognized by self-HLA restricted T lymphocytes (see Chapter 2 ). Recognition of nonself peptides in the context of self-HLA (i.e., altered self) is the function of the T cell antigen receptor and elicits a powerful immune response. The extensive polymorphism of HLA has evolved to enable efficient binding of peptides from the vast array of potentially pathogenic organisms that invade and colonize our bodies. Therefore the evolutionary pressures to develop and maintain diversity vary with time and geographic area. As a consequence, HLA has adapted differently according to geographic region and ethnic group, and HLA phenotypes differ across populations throughout the world.
HLA Genes and Their Products
The HLA system is a complex multigene family consisting of more than 10 loci. HLA types are codominantly inherited on a maternal and paternal haplotype and transmitted as a single Mendelian trait ( Fig. 10.1 ), and therefore an individual can express two alleles at each locus. The genes encoding HLA and their corresponding glycoprotein products are divided into two classes according to their biochemical and functional properties: HLA class I and HLA class II.

HLA Class I
HLA class I genes span two megabases at the telomeric end of the 6p21.3 region of chromosome 6. This region encodes the classical “transplantation antigens” (HLA-A, -B, and -C) that are expressed on virtually all nucleated cells. Genes of the HLA class I loci encode the 44 kD heavy chains which associate with intracellular peptides present within the cytoplasm. The tertiary structure is stabilized on the cell surface by noncovalent association with β 2 -microglobulin, a nonpolymorphic 12 kD protein encoded on chromosome 15. The heavy chain consists of three extracellular immunoglobulin-like domains (α1, α2, α3), a hydrophobic transmembrane region, and a cytoplasmic tail. The two extracellular domains distal to the cell membrane (α1 and α2) are highly polymorphic and fold to form a peptide-binding cleft consisting of eight strands forming an antiparallel beta pleated sheet, overlaid by two alpha helices. The cleft accommodates peptides of 8 to 10 amino acids in length, mostly derived from “endogenous” proteins present within the cell cytoplasm. The major areas of amino acid polymorphism line the sides and base of the cleft and thereby govern the peptide-binding repertoire of the HLA molecule. In contrast, the α3 domain (proximal to the cell membrane) is highly conserved and acts as a ligand for CD8 expressed on T lymphocytes. This interaction confers HLA class I restriction on CD8 positive T lymphocytes, which have a predominantly cytotoxic function and form the basis for cellular immunity to intracellular pathogens such as viruses.
There are other class I loci and knowledge about their expression and function has emerged ( Table 10.1 ). HLA-H, -J, -K, and -L are pseudogenes and HLA-N, -P, -S, -T, -U, -V, -W, -X, -Y, and -Z are gene fragments that are not transcribed or translated. HLA-G is expressed on placental trophoblast cells, implicating a possible involvement in fetal–maternal development. HLA-E, -F, and -G have limited polymorphism and are known to act as ligands for natural killer (NK) cell inhibitory receptors (e.g., CD94). These loci may prove to be important in certain experimental xenograft models and in bone marrow transplantation (where NK cells are involved in the rejection process), but their clinical relevance in solid-organ transplantation has not been firmly established. There is, however, an emerging role for these molecules in innate immunity to persistent viruses such as cytomegalovirus (CMV) and they may prove to have an important role in post transplant viral defense.
Name | Molecular Characteristics |
---|---|
HLA-A | Class I α-chain |
HLA-B | Class I α-chain |
HLA-C | Class I α-chain |
HLA-E | Associated with Class I 6.2kB Hind III fragment |
HLA-F | Associated with Class I 5.4kB Hind III fragment |
HLA-G | Associated with Class I 6.0kB Hind III fragment |
HLA-H | Class I pseudogene |
HLA-J | Class I pseudogene |
HLA-K | Class I pseudogene |
HLA-L | Class I pseudogene |
HLA-N | Class I gene fragment |
HLA-P | Class I gene fragment |
HLA-S | Class I gene fragment |
HLA-T | Class I gene fragment |
HLA-U | Class I gene fragment |
HLA-V | Class I gene fragment |
HLA-W | Class I gene fragment |
HLA-X | Class I gene fragment |
HLA-Y | Class I gene fragment |
HLA-Z | Class I gene fragment (located in the HLA class II region) |
HLA-DRA | DR α-chain |
HLA-DRB1 | DR β 1 -chain determining specificities DR1 to DR18 |
HLA-DRB2 | Pseudogene with DR β-like sequences |
HLA-DRB3 | DR β 3 -chain determining DR52 found on DR17, DR18, DR11, DR12, DR13, and DR14 haplotypes |
HLA-DRB4 | DR β 4 -chain determining DR53 found on DR4, DR7, DR9 haplotypes |
HLA-DRB5 | DR β 5 -chain determining DR51 found on DR15 and DR16 haplotypes |
HLA-DRB6 | DRB pseudogene found on DR1, DR2, and DR10 haplotypes |
HLA-DRB7 | DRB pseudogene found on DR4, DR7, and DR9 haplotypes |
HLA-DRB8 | DRB pseudogene found on DR4, DR7, and DR9 haplotypes |
HLA-DRB9 | DRB pseudogene, probably found on all haplotypes |
HLA-DQA1 | DQ α-chain |
HLA-DQB1 | DQ β-chain determining specificities DQ1 to DQ9 |
HLA-DQA2 | DQ α-chain-related sequence, not known to be expressed |
HLA-DQB2 | DQ β-chain-related sequence, not known to be expressed |
HLA-DQB3 | DQ β-chain-related sequence, not known to be expressed |
HLA-DOA | DO α-chain |
HLA-DOB | DO β-chain |
HLA-DMA | DM α-chain |
HLA-DMB | DM β-chain |
HLA-DPA1 | DP α-chain |
HLA-DPB1 | DP β-chain |
HLA-DPA2 | DP α-chain-related pseudogene |
HLA-DPB2 | DP β-chain-related pseudogene |
HLA-DPA3 | DP α-chain-related pseudogene |
HLA Class II
The HLA class II region consists of three main loci: HLA-DR, -DQ, and -DP. The glycoprotein products are heterodimers with noncovalently associated alpha and beta chains of molecular weight approximately 33 and 28 kD, respectively. Both chains consist of two extracellular immunoglobulin-like domains, a transmembrane region and a cytoplasmic tail. The membrane distal domains α1 and β1 form a peptide-binding cleft similar to, but less rigid than that of HLA class I, accommodating peptides of 10 to 20 amino acids derived predominantly from ingested (endocytosed or phagocytosed) extracellular (exogenous) proteins. The β1 domains of HLA-DR, -DQ, and -DP are highly polymorphic and govern the peptide-binding repertoire. They are constitutively expressed on cells with immune function such as B lymphocytes, activated T lymphocytes and antigen-presenting cells (monocytes, macrophages, and cells of dendritic lineage). HLA class II expression can be induced on most cell types during inflammatory responses (including allograft rejection) by cytokines such as gamma-interferon and tumor necrosis factor alpha. The conserved membrane proximal domain associates with CD4 on T lymphocytes with predominately helper/inducer function and thereby confers HLA class II restriction and forms the basis of cellular and humoral immunity to circulating pathogens such as bacteria.
HLA Polymorphism and Nomenclature
Early investigations into HLA polymorphism used relatively crude alloantisera able to distinguish only a limited number of antigens. Nearly half a century later, these simple techniques have been superseded by molecular methods capable of resolving HLA variants at the DNA sequence level and identifying single amino acid polymorphisms that are indistinguishable by serology. For example, there are currently 19 HLA-DR specificities defined by serologic methods, compared with more than 2000 HLA-DRB sequence variants (alleles) detected using DNA-based typing methods. The number of newly defined alleles identified is still increasing and has now surpassed even the highest expectations of the early pioneers.
Concomitant with the ever-increasing complexity of the HLA region, a nomenclature system has been developed to accurately assign HLA loci and their alleles. This nomenclature system encompasses the methodology (serology, biochemistry, and DNA sequencing) and level to which the HLA genes and their products have been resolved. The nomenclature is complex and, to those outside the field, can appear confusing.
Resolution of HLA Typing Methods
Serologically based HLA typing uses alloantisera and monoclonal antibodies that bind to tertiary epitopes of the cell surface HLA glycoproteins. There is a high degree of sequence homology between HLA specificities and identical amino acid sequence motifs (epitopes) are often shared between groups of antigens.
The degree of HLA compatibility between transplant donors and recipients can be considered at many different levels of resolution, depending on the HLA typing methodology ( Table 10.2 ). This can range from matching for serologically defined epitopes to matching for single amino acid differences detected through high-resolution DNA sequence-based methods (allele matching).
HLA Typing Resolution | Method |
---|---|
HLA allele matching | High-resolution (2-field) DNA sequence-based typing a |
Split HLA specificity matching | Serology and low resolution (generic) DNA typing b |
Broad HLA specificity matching | Serology and low resolution (generic) DNA typing |
HLA-B, -DR matching | Serology and low resolution (generic) DNA typing |
Epitope matching | Serologically defined cross-reactive groups |
Serologically defined motifs/determinants | |
Single amino acid residues | |
Linear peptides and conformational epitopes | |
Supertypic antigen matching | |
Triplet/Eplet amino acid mismatches (HLAMatchmaker) |
a High-resolution DNA typing can be translated into low-resolution serologic equivalents (allele families).
b Low resolution HLA typing by PCR utilizes DNA primers designed to identify polymorphisms at a level comparable to serology.
World Health Organization (WHO) Nomenclature for HLA
HLA genes and their polymorphic products have now been characterized and cloned and have been given official designations using the following principles. The genes are prefixed by the letters HLA followed by the loci or region, for example, HLA-A, HLA-B, or HLA-D. The HLA-D region has several subregions denoted HLA-DR, -DQ, -DP, -DO, and -DM (see Fig. 10.1 ). These are followed by the letters A or B to define the gene encoding the alpha and beta chain gene product of that subregion, respectively (e.g., HLA-DRB genes code for the DRβchain protein product). Where there is more than one A or B gene within a subregion, a corresponding number is given (e.g., HLA-DRB1; see Fig. 10.1 and Table 10.1 ).
A new nomenclature system was launched by the WHO in 2010 to accommodate the growing number of HLA alleles being discovered. In this system each allele is uniquely identified by up to four sets of digits separated by colons (termed fields), prefixed by an asterisk (∗). The digits in the first field usually correlate with the serologic specificity, for instance HLA-B∗27 correlates with the serologic specificity HLA-B27. However, for most serologically defined antigens there is further polymorphism detectable at the DNA and amino acid sequence level. The second field denotes the subtype, listed in the order the alleles were discovered. Alleles with different numbers at this level have nucleotide substitutions that alter the amino acid sequence (e.g., HLA-B∗27:01, HLA-B∗27:02) and so forth. The third field indicates synonymous substitutions within the coding region (i.e., there is no change the amino acid sequence of the expressed protein) and the fourth field indicates polymorphism in noncoding regions.
Some alleles or genes contain sequence defects preventing normal antigen expression at the cell surface. Nonexpressed alleles (null alleles) are indicated using the suffix “N” (e.g., HLA-DRB4∗01:03:01N) whereas alleles with low expression or soluble (secreted) alleles carry the suffix “L” or “S,” respectively. The suffix “C” indicates a protein detected within the cytoplasm and not on the cell surface; “A” aberrant expression, where there is doubt whether the protein is expressed; and “Q,” questionable expression, where a given mutation has been previously shown to affect expression, but the level of expression has not been confirmed for the particular allele.
The HLA-DR and HLA-DP alpha chains have only limited polymorphism and therefore the HLA-DRB1 or -DPB1 allele (which code for the main polymorphic amino acid determinants present on the beta chain) is usually annotated alone. In contrast both the HLA-DQ alpha and beta chains are polymorphic. To describe one of these alleles precisely, definition of both the A and B alleles may be required (e.g., HLA-DQA1∗01:01 and DQB1∗05:01). Although the alpha and beta chain protein products of the A and B gene pairs associate preferentially, there is also the possibility of the formation of novel hybrid molecules. A complete list of recognized HLA genes and their expressed products can be found at www.bmdw.org (Bone Marrow Donors Worldwide; HLA information).
Extended HLA Haplotypes
The HLA region displays strong linkage disequilibrium whereby certain HLA alleles are inherited together as a conserved HLA haplotype. Therefore extended HLA haplotypes involving HLA-A, -B, -C, -DR, and -DQ commonly exist within and between ethnic groups. This greatly improves the probability of finding an HLA-matched unrelated donor as common HLA haplotypes are frequently found within a population (e.g., HLA-A∗01:01, -B∗08:01, -C∗07:01, -DRB1∗03:01, -DRB3∗01:01, -DQA1∗05:01, -DQB1∗02:01). There is only relatively weak linkage centromeric to HLA-DQ because of a recombination “hot-spot” between HLA-DQ and -DP.
HLA on the Web
Information concerning the HLA system is rapidly expanding and articles such as this are always out of date by the time they go to print. However, there are a number of Internet websites with useful links that are regularly updated. These provide contemporary articles and information concerning HLA genes, nomenclature, polymorphism, DNA and amino acid sequences for both lay and professional readers:
HLA Matching
It was more than 40 years ago that HLA matching between donor and recipient was found to be associated with better transplant and patient survival. Matching for the class I HLA-A and -B antigens influenced survival, but matching for the class II HLA-DR antigens was shown to have the most powerful effect. A beneficial effect of HLA matching on graft survival was demonstrated in analyses of large data sets and national and international databases.
Over the years there has been an overall improvement in transplant survival and a decrease in the survival advantage conferred by HLA matching. The improvement can be attributed to a number of factors, but one of the most powerful is advancements in the effectiveness of immunosuppression. This was clearly demonstrated in a local comparison of transplant survival in patients receiving azathioprine and prednisolone, cyclosporine and prednisolone, and triple therapy (cyclosporine, azathioprine, prednisolone) where 1-year transplant survival rates were 65%, 69%, and 81%, respectively. In this analysis HLA-DR compatibility still had a marked effect on the posttransplant clinical course, with an increased incidence of rejection in HLA-DR mismatched grafts, the socioeconomic effects of which were increased use of immunosuppressive drugs, longer hospital stays, and higher 3-month creatinine levels.
Despite further improvements in immunosuppression and patient management, a beneficial effect of HLA matching is still apparent in data from recent transplants published from the Collaborative Transplant Study ( Fig. 10.2 ) and from the United Network of Organ Sharing (UNOS). The UNOS data revealed a linear relationship between mismatches at HLA-A, -B, -DR, and allograft survival, independent of HLA locus, in 189,141 transplants performed between 1987 and 2013. Although the transplants analyzed were performed over a long time period, the HLA effect was present in the transplants performed in recent periods.

In analyzing the effect of HLA on transplant outcome, it is important that other factors known to have a strong influence on outcome are taken in account. In a rigorous multivariate analysis of factors influencing the outcome of primary deceased donor transplants in a cohort of transplants performed in the UK between 1986 and 1993, the year of transplant, donor and recipient age, waiting time to transplant, diabetes in the recipient, donor cause of death, exchange of kidneys, cold ischemia time, and HLA mismatching were found to influence transplant survival (death with function treated as failure). The best transplant survival was achieved in transplants with no mismatches at HLA-A, -B, and -DR (“000” HLA-A, -B, -DR mismatch grade). Other well-matched transplants, termed “favorably matched transplants,” with a maximum of one HLA-A and one HLA-B antigen mismatched in the absence of mismatches at HLA-DR (110, 100, 010 mismatch grades) had a significantly improved survival over transplants of all other match grades. An analysis of factors influencing the long-term outcome of these transplants revealed that for patients with transplants functioning after 6 years, only older donor age and diabetes had a significant detrimental influence on survival.
The influence of HLA mismatch on outcome of first deceased donor transplant was investigated in a cohort of patients, transplanted in the UK between 1995 and 2001. As a result of the allocation policy the recent transplants were significantly better matched than the previously analyzed cohort (1986–1993), where 46% transplants were 0-DR mismatched and 10% had 2-DR mismatches, compared with 60% 0-DR mismatched and only 3% 2-DR mismatches in the 1995 to 2001 cohort. In a multivariate analysis there was no effect of HLA-A mismatching, but a significant effect of two mismatches at HLA-B and an incremental effect of mismatching at HLA-DR. In recent multivariate analyses of the outcome of 7837 adult patients transplanted in the UK between 2009 to 2014, there is still a significant beneficial effect of HLA matching on graft outcome at 1 and 5 years posttransplant.
There have been several publications from the Collaborative Transplant Study revealing associations between HLA mismatching and clinical events posttransplant. Increasing numbers of HLA mismatches were shown to be significantly associated with an increased requirement for antirejection treatment and with the cumulative incidence of death with a functioning graft resulting from cardiovascular disease or infection, but not from malignant neoplasm. The number of HLA-DR mismatches was shown to be associated with increased incidence of non-Hodgkin’s lymphoma and hip fractures. It is possible that these associations result from the higher levels of immunosuppression used in the management of poorly HLA-matched transplants.
In solid-organ transplantation the effects of HLA matching reported are generally based on matching at the HLA-A, -B, and -DR loci. The effect of matching other HLA loci has been analyzed, but demonstrating an independent effect is difficult because of linkage disequilibrium.
Matching for HLA-DQ has been variously reported as having either a beneficial effect or no effect on transplant outcome However, in a recent analysis of data from the Australia and New Zealand Dialysis and Transplant Registry, HLA-DQ mismatches were found to be associated with an increased incidence of acute rejection, independent of HLA-A, -B, -DR mismatches. This effect was further increased when donors and recipients were mismatched for HLA-DR. Registry analysis has shown that HLA-DPB matching has an effect on the transplant survival of regrafts, but not of first transplants. It is possible that this effect results from matching for certain immunogenic HLA-DPB epitopes and undetected immunologic priming against donor-DP mismatches of the failed graft.
The definition of an HLA match between donors and recipients can be considered at different levels depending on the resolution of the HLA type and consideration of areas of similarity and differences in the HLA molecules. The influence of compatibility for the broad serologic specificities, serologic split specificities, epitopes common within serologic cross reactive groups (CREGs), single amino acid differences, or epitopes has been considered in deceased donor renal transplantation, and matching at these various levels has been reported to be associated with improved outcomes.
There is now considerable interest in epitopes on the HLA molecules and the opportunity to reduce the immunogenicity of the transplant by matching donor and recipient for immunodominant epitopes, with the aim of reducing the potential for the immune response and the development of donor-specific HLA antibodies after transplantation. This topic will be covered in a later section.
HLA-Specific Allosensitization
The single most important function of an H&I laboratory is to detect recipient immunologic priming against foreign HLA (allosensitization) and to avoid transplantation of recipients with preexisting donor HLA-specific antibodies (DSA). After an encounter with HLA alloantigens, naïve T and B lymphocytes elicit immune effector functions to isolate and destroy allogeneic tissue. In the context of transplantation, however, calcineurin-based immunosuppressive regimes are effective in blocking naïve T and B cell activation and, in the modern era, early allograft loss in nonsensitized recipients due to irreversible allograft rejection is uncommon. In contrast, memory T and B cells that have been primed by previous exposure to foreign HLA are often resistant to conventional immunosuppressive therapy and are likely to cause irreversible cellular and/or humoral allograft rejection. Although HLA matching is effective in avoiding transplantation between “incompatible” allosensitized donor–recipient pairs, because of the high level of heterogeneity between HLA types within and between populations, most organ transplants between genetically unrelated individuals are mismatched at one or more HLA loci.
HLA-specific allosensitization can occur at both the T cell (cellular) and B cell (humoral) level but current technology to detect alloreactive memory T and B cells is limited to translational research in specialist laboratories. In the absence of large scale routinely applicable assays to detect cellular sensitization, allosensitization is determined indirectly by the presence of circulating IgG HLA-specific antibodies in recipient serum. HLA-specific IgG is secreted by long-lived bone marrow resident plasma cells that are formed after B cell/alloantigen engagement and cognate interaction with CD4 (helper) T cells. This results in B cell maturation, clonal expansion, immunoglobulin class switch (from IgM to IgG), and the formation of antigen-specific memory T and B cells. On repeat exposure to the same antigen, memory T and B cells elicit a rapid and aggressive immune response with potential to cause irrevocable graft damage. Therefore circulating IgG HLA-specific antibodies are an effective but incomplete surrogate for detecting alloreactive T and/or B cell memory.
The most common route of patient allosensitization is by exposure to foreign (nonself) HLA after blood transfusion, pregnancy, and/or a previous allograft. Approximately 20% of pregnant women produce HLA-specific antibodies to paternally inherited fetal HLA antigens and pregnancy followed by blood transfusion is a potent stimulus for the formation of high-level and broadly reactive IgG HLA-specific antibodies. Similarly, HLA-specific antibody formation is common after a failed allograft, and many such patients become “highly sensitized” with antibodies that react to HLA alloantigens present in the majority of the potential donor population. The extent of sensitization in patients awaiting a second or subsequent transplant is related to the number of donor-recipient HLA mismatches and continuation or withdrawal of maintenance immunosuppression after graft failure and return to the transplant waiting list. Therefore, to avoid the development of high levels of sensitization, clinical management of patients with a failing graft should include consideration of the risks associated with maintaining immunosuppression together with future options and a timescale for repeat transplantation. In addition to these classical routes of allosensitization there is increasing awareness of naturally occurring HLA-specific antibodies that may result from cross-reactivity with infectious agents or autologous tissue damage. Such “idiopathic” antibodies are reactive with specific epitopes expressed on denatured HLA proteins (considered clinically benign) or native (conformationally folded) HLA proteins that have potential to cause graft rejection.
HLA-Specific Antibody Detection and Characterization
Over the past 15 years new technologies for the detection and characterization of HLA-specific antibodies have been developed enabling a more accurate assessment of complex antibody populations present in patient serum and comprehensive elucidation of a patient’s sensitization profile. The available technologies are outlined next.
Complement-Dependent Lymphocytotoxicity
The complement-dependent lymphocytotoxicity (CDC) test was the first technique applied routinely for HLA-specific antibody screening; it is also used for the donor lymphocyte crossmatch test. In this assay, lymphocyte target cells are used to detect complement-fixing IgM and IgG antibodies present in patient’s serum, as indicated by cell lysis after the addition of rabbit complement ( Fig. 10.3 ). IgM antibodies can be differentiated from IgG antibodies by pretreatment of patient serum with dithiothreitol (DTT) that reduces the disulfide bonds in the IgM pentamer, but leaves IgG relatively intact. Patient serum is tested against lymphocyte panels obtained from volunteer blood donors that can either be “random” or alternatively “selected” to represent the HLA types in the potential organ donor population (termed panel reactive antibodies ; PRA). The CDC assay is used to indicate the presence (and provide limited specificity analysis) of recipient lymphocytotoxic antibodies with potential to cause a positive donor lymphocyte crossmatch.

There are a number of limitations of the CDC technique. Only complement-fixing antibodies are detected, viable donor lymphocytes are required, and the assay sensitivity is dependent on the particular batch of rabbit complement used. Characterization of complex antibody profiles in patient serum is limited, and both HLA and non-HLA lymphocytotoxic antibodies are detected. Although the use of DTT can differentiate IgM from IgG antibodies, this does not indicate the specificity of the antibody and potentially clinically relevant weak IgG HLA-specific antibodies may also be rendered negative after the addition of DTT to patient serum. Reactivity resulting from an IgM HLA-specific antibody is indistinguishable from reactivity of an IgM non-HLA-specific (often auto-reactive) antibody. However, such antibodies are frequently weak or nonreactive with lymphocytes from patients with B cell chronic lymphatic leukemia (CLL), and therefore including these cells in the screening panel can be useful in elucidating a patient’s antibody profile.
There have been a number of approaches used to increase the sensitivity and specificity of the CDC test to detect low-level antibodies that are potentially harmful. These include increasing the incubation times, the wash (Amos) technique and augmentation with antihuman globulin (AHG). In the Amos technique, unbound antibody is washed from the cell suspension before the addition of rabbit complement, thus removing the anticomplementary factors and low-affinity IgM antibodies in the serum to preferentially detect clinically relevant IgG antibodies. In the AHG augmentation CDC test, antikappa light chain is added to the washed cells before the addition of complement, thus increasing assay sensitivity.
Solid-Phase Binding Assays for Hla-Specific Antibody Detection and Specification
It is now recognized that humoral rejection caused by HLA class I- and/or class II-specific antibodies present at levels below the detection threshold of the CDC assay is a major cause of allograft rejection and year-on-year graft attrition. This stimulated the development of new technology (solid-phase binding assays; SPA) that provide increased test sensitivity (to detect low-level antibodies) and specificity (to identify antibodies likely to damage a transplanted organ). Various commercially available SPA kits are all based on the common principle—incubation of patient serum with purified HLA protein bound to a polystyrene surface and detection of HLA-specific antibody binding using a fluorochrome- or enzyme-labeled antihuman IgG conjugate.
The first SPA techniques used for HLA-specific antibody detection and specification were enzyme-linked immunosorbent assay (ELISA) and flow cytometry, but more recently these have been largely superseded by “Luminex” technology that uses purified HLA proteins bound to polystyrene microbeads ( Fig. 10.4 ). The microbeads are impregnated with two fluorescent dyes in different ratios to enable differentiation of up to 100 different bead populations using a dedicated dual-laser flow cytometer (Luminex platform). Each bead population is coated with purified HLA proteins of multiple or single HLA class I or HLA class II alleles which allow comprehensive detection and specification of HLA-A, -B, -C, and -DR, -DQ, and -DP specific antibodies, respectively. The antigen-coated beads are incubated with patient serum and HLA-specific antibody binding is detected using a fluorescent-labeled antihuman-globulin antibody. Luminex single-antigen beads (SAB) enable rapid and simultaneous elucidation of multiple antibody populations in a patient’s serum and, for the first time, facilitates accurate specification of complex antibody profiles in highly sensitized patients. Although commercially available Luminex-based HLA-specific antibody detection and specification kits are currently licensed for qualitative use only, the semiquantitative readout (Median Fluorescence Intensity; MFI) has been used to indicate antibody levels that form an important function in informing a pretransplant immunologic risk assessment. However, the Luminex assay is subject to a number of in vitro artifacts, and interpretation of MFI values requires caution and expert knowledge. SAB express variable levels of denatured HLA to which antibody binding is common, giving rise to a false-positive DSA. In addition, inhibitory factors in patient sera may block HLA-specific antibody detection and give rise to a misleadingly low assessment of DSA. Such inhibition, however, is easily overcome by serum dilution, heat inactivation, or the addition of EDTA.
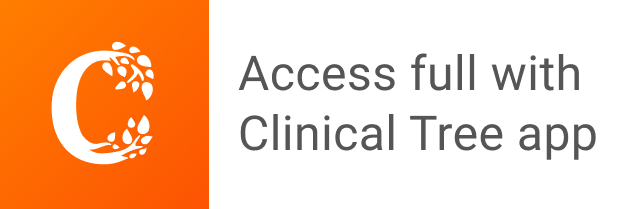