Fig. 7.1
(a) Schematic representation of the HBV virions, HDV virions, and HBV subviral particles (SVPs). The HBV DNA genome and the HDV RNA genomes are represented as circular molecules. Open reading frames for the envelope proteins (env), polymerase (pol), capsid (core), and x proteins are indicated on the HBV genome. HDAg ORF is indicated on the HDV genomic strand. S-HBsAg small HBV envelope protein, M-HBsAg middle HBV envelope protein, L-HBsAg large HBV envelope protein, L-HDAg large HDAg protein, S-HDAg small HDAg protein. The diameter of HBV virions, HDV virions, and SVPs is indicated in nanometers (nm). (b) Schematic representation of the HBV and HDV replication cycles. The HBV and HDV replication cycles are indicated in pink and blue, respectively. IC intermediate compartment, ER endoplasmic reticulum, MVB multivesicular bodies, g genomic HDV RNA, ag antigenomic HDV RNA
In humans, HDV infection occurs either as a coinfection when individuals are simultaneously infected with both HBV and HDV, or as a superinfection when HBV chronic carriers become infected with HDV. Acute HDV superinfection leads more often to fulminant hepatitis and liver failure than does acute infection with HBV alone. In all cases, HDV can cause severe liver diseases , and in most HBV carriers, superinfection with HDV becomes chronic. Liver damage also occurs more rapidly in chronically HBV/HDV infected patients as compared to HBV carriers (See Chap. 14 for details). It is estimated that 5–10 % of the more than 240 million HBV chronic carriers worldwide are coinfected with HDV [17]. HDV is present in many different countries worldwide, and it displays an extended genetic variability classified into eight genotypes [18]. Although HDV infection is declining in some endemic regions, it is not disappearing, in particular in Europe, as a result of immigration from high endemic countries [19, 20]. Unfortunately, the possibilities for treating chronic HDV infection are limited to interferon, a therapy characterized by a low rate of sustained virological response [21, 22]. Therefore, there is actually an increasing need for the development of novel antiviral strategies against HDV, which will hopefully emerge from studies conducted to better understand the molecular interplay between HDV and its helper HBV.
The HDV Genome and Its Replication
HDV displays many unique features, among which the obligate relationship with HBV and the structure of its genome. The HDV RNA is approximately 1700 nucleotides in size and the smallest genome of viruses known to infect humans [23]. Both genomic RNA, and its replication intermediate, the antigenomic species, are circular molecules that form an unbranched, quasi-double-stranded species, also referred to as rod-like structure due to a nucleotide base pairing of approximately 70 %. The circular conformation and self-annealing properties are thought to confer resistance to host nucleases. The rod-like structure includes alternates of base paired helices and small internal loops or bulges of single stranded sequences, which are required for efficient replication [24], interaction with the HDAg proteins, and assembly of RNP complexes [10, 25–28]. A single protein is encoded by an ORF present on the antigenomic RNA strand [29]. The viral protein bears HDAg and is detected under two isoforms referred to as small-HDAg (S-HDAg) of 195 amino acids in length, and large-HDAg (L-HDAg) that differ from S-HDAg by 19 additional amino acid residues at the C-terminus. These isoforms are the result of an RNA editing event that occurs on a replication intermediate of the viral genome and is copied onto the HDAg mRNA [30]. The rod-like structure of the HDV genome can be divided in two domains (Fig. 7.2), a viroid-like domain with a high degree of sequence conservation and a larger domain including the HDAg ORF and its complementary sequence in the rod structure [31].


Fig. 7.2
The different forms of HDV RNAs in HDV-replicating cells. Genomic, antigenomic and messenger HDV RNAs are represented. The position of the tips of the rod-like structures is indicated. The HDAg ORF is indicated. Arrows on the RNA strands indicate the 5′–3′ direction
After the initial sequencing of the HDV genotype-I (HDV-I) genome [23], seven additional genotypes have been identified [18], the nucleotide sequences of which display a surprisingly extensive divergence. HDV-III, a genotype specific to South America, is the most distant to other genotypes, nearing 40 % divergence with HDV-I. It is also associated to the most divergent helper HBV genotype (genotype-F), and to the most severe HDV-associated disease [32]. The majority of the molecular studies have been conducted with HDV-I, the prototype and ubiquitous genotype worldwide.
It was assumed that the replication mechanisms of HDV and plant viroids would be identical [33], since their RNAs displayed similarities [8, 23]. Experimental approaches to study HDV replication were therefore chosen based on the models already established for viroids [34, 35], including the initiation of HDV RNA replication by transfection of mammalian cells with cloned HDV cDNA. HDV RNA was observed to replicate in the nucleus, as efficiently as viroids in plant cell nuclei or chloroplasts , in the absence or presence of HBV [34, 36]. In subsequent studies, there were indications that the single-stranded circular genomic (or antigenomic) HDV RNA was used as a template for a rolling-circle mechanism of antigenomic (or genomic) RNA synthesis. In its principle, the rolling-circle mechanism should lead to synthesis of multimeric antigenomic RNA from the circular genomic template, but such a multimer does not accumulate because of its cleavage into monomers by an autocatalytic activity—defined as ribozyme —soon after synthesis. The linear monomer product is then ligated on itself to form a circular antigenomic molecule, which in turn serves as a template for the synthesis of genomic RNAs through the same rolling circle model [37]. Unlike viroids, the HDV RNA replication cycle includes the synthesis of a messenger RNA of antigenomic polarity for translation to HDAg proteins that fulfill multiple functions in the virus life cycle [38]. Although deprived of RNA polymerase activity, S-HDAg is required for HDV RNA replication, while L-HDAg inhibits replication and mediates assembly of HDV virions. Thus, HDV must use a host RNA polymerase to replicate its genome, as do viroids in plants.
HDV RNA Synthesis by Host RNA Polymerase
RNA-dependent RNA polymerase (RdRP) activity has long been considered absent in mammalian cells, but there is now clear evidence for its existence. For instance, the detection of intracellular mirror-spliced antisense transcripts that are reverse complement of spliced mRNAs was indicative of direct synthesis from an RNA template [39]. Many RNA viruses replicate their genome by encoding an RdRP for synthesis of both new genomes and viral mRNAs. However, in the case of Retroviruses, it is the host RNA Polymerase II (Pol II) that acts as a replicase to generate multiple viral genome copies from the provirus integrated into the host DNA. Pol II is also the enzyme that indirectly amplifies the HBV genome by transcribing the latter into multiple copies of pre-genomic RNA. For HDV RNA replication, several studies have shown that Pol II, which normally transcribes DNA templates into RNA, is somehow diverted to use the circular HDV RNA species as a template [40, 41]. Pol II would therefore act as a replicase for both HDV and HBV genomes.
The studies that identified Pol II as the enzyme responsible for HDV RNA synthesis, have been conducted in cell cultures in which there was no possibility of transcription of HDV RNA from DNA [42, 43]. In this setting, synthesis of genomic and antigenomic HDV RNAs, was prevented by the Pol II-specific inhibitor alpha-amanitin . HDV RNA replication was shown to colocalize with HDAg, Pol II, and the splicing factor SC35, in structures of the nucleoplasm referred to as speckles or transcription factories , whereas in the absence of HDV replication, HDAg would accumulate in the nucleoli [43]. When HDAg was artificially targeted to the nucleoli, initiation of genomic RNA synthesis was abolished, whereas antigenomic RNA synthesis was not affected. Forcing the release of S-HDAg from the nucleoli with actinomycin D would restore genomic RNA synthesis and the interaction between S-HDAg and Pol II, suggesting that synthesis of genomic RNA and HDAg mRNA would take place in the nucleoplasm, and antigenomic RNA in the nucleoli [44]. Yet, there is still controversy as to the implication of host RNA polymerases other than Pol II, in HDV RNA-directed synthesis, based on the observation that in cells transfected with genomic HDV RNA, synthesis of antigenomic species was recorded in the presence of 100 μg/ml of alpha-amanitin, whereas synthesis of genomic RNA was inhibited at 2.5 μg/ml [45]. It suggested to the authors that Pol I, an enzyme involved in the synthesis of ribosomal RNAs, rather than Pol II, could carry out antigenomic RNA synthesis [46].
Several attempts were made to reconstitute HDV RNA synthesis in vitro. For instance, it was shown that S-HDAg could bind directly to Pol II and stimulate transcription by displacing a negative elongation factor (NELF) [47]; S-HDAg could also interact with the clamp of Pol II, to ensure transcription fidelity [48]. Using purified Pol II, NTPs and an HDV antigenomic template scaffold, RNA-dependent Pol II activity appeared slower and less processive than its DNA-dependent activity [49]. Abrahem and colleagues [50], observed that an active Pol II pre-initiation complex could form on the HDV RNA with direct binding of the TATA-binding protein . The promoter sequence for synthesis of antigenomic RNA was proposed to map to a 29-nucleotide region on the genomic strand (nucleotides 1650–1679), and the one for genomic synthesis, to a short region on the antigenomic species surrounding position 1679 [51, 52]. Pol II is assumed to recognize the quasi double-stranded structures of these hairpins as promoters. In cells undergoing active HDV RNA replication, two 5′-capped HDV RNA species of 18-25-nucleotides in length were identified, including one corresponding to the 5′ end of HDAg mRNA, which could interact with HDAg and Pol II. These small RNAs would act as initiators of RNA polymerization [53].
HDV Ribozymes
One characteristic of the rolling-circle mechanism used by viroids to replicate is the synthesis of longer-than-unit-length products from a circular template, which are processed to monomers by an autocatalytic, or ribozyme, activity. The linear monomers are then converted to circular molecules by self-ligation—or the action of a cellular ligase—to serve as templates for a second round of the rolling-circle process . HDV ribozymes consist in RNA sequences present on both genomic and antigenomic RNA species with self-cleavage activity and similar structural features, but they differ in size, sequence and secondary structure from hammerhead or hairpin ribozymes of viroids or satellites of plant viruses [54]. The reaction catalyzed by HDV ribozymes is a trans-esterification that converts a 3′–5′ phosphodiester bond to a 2′–3′ cyclic monophosphate group and a 5′ hydroxyl group. It is modulated by divalent metal ions, and easily observed in vitro in the absence of cellular proteins. The minimal size of the HDV ribozymes for self-cleavage in vitro was established at approximately 85 nucleotides and, a single nucleotide, 5′ to the cleavage site, is sufficient for cleavage . Considerable advances have been made recently, including X-ray structural data [55] providing details about the mechanism of HDV ribozyme activity (for review, see ref. [56]).
In an HDV-infected cell, self-cleavage and ligation are probably regulated to control the rate of genome replication and best accommodate the HDV life cycle. For instance, since antigenomic and messenger HDV RNAs are both synthesized from the same promoter on the genomic strand, it is expected that on the nascent antigenomic transcript, the ribozyme sequence, located just 3′ of the polyadenylation site, interferes with polyadenylation [57]. However, since mRNA and antigenomic RNA are transcribed in separate nuclear compartments, interference might be limited [45].
Interestingly, following the discovery of HDV, many HDV-like ribozymes have been identified in different branches of life and found to play a role in a variety of biological events, including retro-transposition in eukaryotes , raising the possibility of HDV RNA originating from the cell transcriptome [58]. Furthermore, recent studies have shown that circular RNA species are relatively abundant in cells [59], leading to the speculation that HDV RNA would have emerged in HBV infected hepatocytes in which viral RNA species were processed to circular forms that became eventually replicative [60]. A cellular origin of HDV has been discussed previously [61], after the identification of the cellular homolog of HDAg, termed delta-interacting protein A (DIPA) . DIPA was then proposed as a possible ancestral protein of HDAg. In this scenario, HDV would have evolved from capture of a cellular transcript by a self-replicating circular RNA. Since HDV is found only in humans, these findings might indicate a recent origin of HDV, with both ribozyme and coding sequence arising from the human transcriptome [62].
HDAg Proteins and RNA Editing
A single ORF on the antigenomic HDV RNA encodes the S- and L-HDAg proteins that differ from each other by 19 additional amino acid residues at the C-terminus of L-HDAg. S-HDAg is essential for RNA replication/accumulation, whereas L-HDAg acts as a potent dominant negative inhibitor of S-HDAg [63] while promoting virion assembly [64].
The origin of the two HDAg isoforms was initially revealed by analyzing the HDV RNA sequences that emerge during the natural course of infection: a heterogeneity at codon 196 in the HDAg ORF was observed, changing from a UAG amber codon to UGG for tryptophan, and respectively corresponding to S-HDAg (195 residues in length) and L-HDAg (214 residues) [65]. The sequence modification was found to occur during the course of infection, leading to the production of S-HDAg at the early phase of HDV RNA replication, and L-HDAg at the late phase of assembly. It was then found to result from an editing event on the full length antigenomic HDV RNA, catalyzed by a cellular adenosine deaminase that acts on RNA (ADAR). This enzyme converts adenosine to inosine, a nucleoside recognized as a guanosine [66]. The small form of ADAR-1 is in charge of editing HDV RNA [67, 68], and it plays a critical role in the regulation of the HDV life replication cycle by controlling the switch between genome amplification and virion assembly (for review, see ref. [30]). Interestingly, there are significant differences in the editing efficiency between HDV genotypes I and III [69].
The HDAg proteins undergo multiple posttranslational modifications to fulfill numerous functions in HDV RNA replication process, RNP assembly and interaction with the HBV envelope proteins [70, 71]. Several of these functions are achieved upon the ability of specific HDAg isoforms to interact with HDV RNA and to localize to particular subcellular compartments. In fact, the RNP and/or HDAg proteins can shuttle between nucleus and cytoplasm , and within the nucleus, between nucleoplasm and nucleoli.
HDAg proteins undergo methylation at Arg-13 [72], acetylation at Lys-72 [73], phosphorylation at Ser-177 [74] and sumoylation at multiple lysine residues [75]. All these posttranslational modifications are thought to alter the HDAg protein conformation, intracellular localization and function in RNA replication mechanism and RNP assembly (for review, see ref. [70]).
HDV Ribonucleoprotein
Replication of the HDV genome by RNA Pol II is very efficient, leading to the accumulation of up to 300,000 copies of genomic HDV RNA per cell, 50,000 copies of the antigenomic species and approximately 1000 copies of a 900-nt-in-length HDAg mRNA (Fig. 7.2). Since extracellular HDV virions contain exclusively genomic RNA, there must be a process for selection of this molecule to assemble RNPs that are released as enveloped virions. All forms of HDV RNA are synthesized in the nucleus, but soon after synthesis, a significant amount of newly synthesized genomic RNA is detected in the cytoplasm [76]. In fact, the majority of intracellular HDAg proteins and genomic RNA are detected in the cytoplasm, whereas most of the antigenomic RNA is retained in the nucleus . The proportion of genomic HDV RNA in the nucleus, compared to cytoplasm, remains relatively constant over time, indicating that genome export from the nucleus would occur continuously [26]. Since nuclear export of genomic RNA appeared resistant to leptomycin B, a cell region maintenance-1 (Crm1)-independent pathway is likely to be involved [76]. This pathway is used for the splicing-dependent export of cellular mRNAs synthesized by Pol II. This is in agreement with HDV RNA synthesis being carried out by Pol II.
HDAg proteins and HDV RNA form a stable RNP complex that adopts a spherical core-like structure with a diameter of 19 nm in HDV virions or within nuclei undergoing HDV replication . Unlike virion-associated RNPs, intracellular RNPs were found to contain both genomic and antigenomic RNAs. The molar ratio of HDAg proteins to HDV RNA was initially estimated at 70 in HDV virions and 30 in nuclear RNPs [10], then at 200 in both virions and intracellular RNPs [26]. More recently, a study based on RNP reconstruction assays estimated that a full-length HDV RNA molecule would associate to 32–40 HDAg proteins [28]. This ratio was in the same range as the early estimates of Ryu and colleagues [10].
To bind to HDAg proteins, the HDV RNA must be structured as an unbranched rod of at least 300 nucleotides that includes stretches of double stranded RNA interspersed with single stranded loops within the rod. According to Lin and colleagues [28], an HDAg multimer of fixed size, would assemble prior to RNA binding. The multimer would adopt the octameric organization that Zuccola and colleagues predicted using HDAg-specific synthetic peptide and X-ray crystallography [77]. These authors proposed that HDAg proteins would form dimers arranged as an antiparallel coiled coil (residues 13–48), and those dimers would further associate to form octamers . Combined with the data reported by Lin and colleagues [28], these results suggest that HDAg would bind HDV RNA as preformed octamers leading to an RPN consisting of one genome and 4–5 octamers.
Within S- and L-HDAg, two arginine-rich motifs (ARMs) , I and II, located in the middle of the protein sequence, were initially thought to mediate binding to HDV RNA [78]. However a recent study demonstrated that ARMs I and II are not required for HDV RNA binding [79]. Instead, RNA binding would be established via multiple domains of the HDAg protein among which the N-terminal region of HDAg would play the prominent role [80, 81].
A functional nuclear export signal (NES) is present at the C-terminal 19-amino acid sequence of L-HDAg [82], however, it remains unclear whether it is required for RNP nuclear export and virion assembly. Since nuclear export of genomic RNA appeared independent of L-HDAg, it is conceivable that genome-containing RNPs would be assembled in the cytoplasm [76].
A CXXQ signal for farnesylation is also present at the L-HDAg C-terminus [83, 84]. This posttranslational modification is required for virion assembly [14, 85]. It is assumed that the farnesyl group serves to anchor the RNP to the membrane of the endoplasmic reticulum (ER), where the envelope proteins are synthesized. In tissue culture, the co-expression of L-HDAg and HBV envelope proteins leads to L-HDAg packaging within HBV subviral particles (SVPs) , but such assembly of L-HDAg-containing SVPs would not occur during the natural course of HDV RNA replication [86].
The 19-amino acid C-terminus of L-HDAg also contains a clathrin box, and L-HDAg is found to colocalize with the HBV envelope proteins and clathrin heavy chain in clathrin-coated vesicles, suggesting the involvement of a clathrin-L-HDAg interaction in the process of virion assembly [87–89]. However, the L-HDAg C-terminal clathrin box is not conserved among HDV genotypes.
Finally, the 19-amino acid C-terminus of L-HDAg bears a motif responsible for binding to the HBV envelope proteins. It includes proline residues that are present at several positions, and important for both NES function and activity of the packaging signal [13, 14]. Surprisingly, beside the CXXQ farnesylation signal sequence and tryptophan at position 196, the 19-amino acid C-terminus sequence of L-HDAg is not well conserved among the different HDV genotypes, except for the presence of at least four proline residues that are dispersed at various positions between W-196 and C-211 [90].
HDV Virion Assembly
The HDV virions are heterogeneous in size with an average diameter of 36 nm. The envelope is similar to that of HBV particles, including cell-derived lipids associated to HBV envelope proteins surrounding the inner HDV RNP [26].
The HBV helper function solely consists in providing HDV the means for propagation. HBV is an enveloped virus of the Hepadnaviridae family , with an icosahedral nucleocapsid that contains a circular, partially double-stranded DNA. Its genome replicates through a mechanism that includes a step of reverse transcription (see Chap. 1 for details). But the distinctive features of the HBV life cycle, essential to HDV propagation, reside in its peculiar, envelope protein-driven budding mechanism. In HBV-infected cells, the three envelope proteins, designated small, middle, and large (S-HBsAg, M-HBsAg, and L-HBsAg, respectively), are produced in amounts exceeding by far the need for HBV virion assembly, and they have the capacity of self-assembly, leading to the secretion of SVPs [91]. S-HBsAg proteins can dimerize and form multimers at the ER membrane through lateral protein–protein interactions , and the resulting aggregates are thought to bud spontaneously into the lumen of a pre-Golgi compartment as empty SVPs. Although the S-HBsAg protein provides the driving force to the budding process , it cannot direct HBV virion assembly because the recruitment of the HBV nucleocapsid is mediated by L-HBsAg. Owing to the overproduction of S-HBsAg and to its capacity for auto-assembly, HBV infectious virion formation is a rather rare event compared to the production of SVPs. As a result, an average infectious serum contains approximately 1012–13 SVPs and only 108–9 virions per ml. In comparison, at the onset of an acute HDV infection, titers of 109–10 HDV virions per ml and a tenfold excess of SVPs are observed. Therefore, HDV particles appear more efficiently assembled and secreted than HBV virions.
The HBV envelope proteins are membrane-spanning glycoproteins that differ from each other by the size of their N-terminal ectodomain [91]. L-HBsAg contains a N-terminal pre-S1, central pre-S2 and C-terminal S domains. M-HBsAg is shorter than L-HBsAg in lacking pre-S1. S-HBsAg only consists of the S domain. Envelope proteins are synthesized at the ER membrane; they form aggregates that bud at a pre-Golgi compartment before egress as SVPs [92]. Assembly of mature HBV virions requires, in addition to S-HBsAg, the presence of L-HBsAg as a matrix protein for nucleocapsid envelopment [93]. Recent findings indicate that HBV virions and SVPs follow distinct pathways for export: the late endosomal multivesicular bodies (MVBs) for budding of HBV virions at intracellular membranes, and an MVB-independent secretory pathway for the release of SVPs [94–96]. The HBV envelope proteins can also package the HDV RNP in the case of HBV/HDV coinfection [2, 12], leading to the assembly of HDV virions. Whether HDV uses the SVPs secretion pathway rather than an MVB-dependent route for export remains unclear.
HDV infection has only been found in humans and solely in association with HBV infection. However, among close members of HBV in the Hepadnaviridae family , the Woodchuck hepatitis virus (WHV) and the Woolly monkey hepatitis B virus (WMHBV) encode viral envelope proteins that are competent for HDV RNP envelopment [3, 97]. These findings have led to the use of woodchuck as a convenient small animal model for HDV, and to the possibility of using primary cultures of hepatocytes from woolly monkey or spider monkey to test for the infectivity of HDV particles pseudotyped with the WMHBV envelope proteins [97]. In contrast to WHV and WMHBV, the most distantly related Hepadnavirus, namely the Duck hepatitis B virus (DHBV) , is unable to assist in HDV propagation. It is due in part to the failure of HDV RNA to replicate in avian cells [98], but also to the inability of the S-DHBsAg envelope protein to package the HDV RNP [99].
A crucial step in the HDV life cycle is the recruitment of the HBV envelope proteins by the RNP in the cytoplasm. L-HDAg exposed at the surface of the RNP must interact with a specific motif of the HBV envelope proteins. Early studies conducted by Chen et al. [100] had shown that a truncation of the C-terminal 50 residues of S-HBsAg was sufficient to abolish envelopment and secretion of co-expressed HDAg proteins. Then, O’Malley and Lazinski [99] demonstrated that S-HBsAg bearing a deletion in the AGL (aas 107–147) was competent for L-HDAg interaction, but defective for virion assembly and release. A deletion of residues 24–28 of the S-HBsAg protein, or the removal of the glycosylation site at position 146 could also impair the maturation of HDV particles while having no effect on SVP secretion. In the two latter cases, the defect in HDV assembly was due to impairment of the mutant envelope to coat the RNP and not to a lack of interaction with L-HDAg. More recently, a major determinant of HDV assembly was identified in the C-terminus of S-HBsAg [13]. Within this domain, single substitutions of tryptophan (Trp) at positions 196, 199 and 201 with alanine or phenylalanine, were permissive to SVP secretion but detrimental to HDV assembly. This was proven to result from the inability of the S-HBsAg mutants to interact with L-HDAg. The Trp-rich domain in S-HBsAg was thus considered as a matrix domain for HDV assembly [13]. A recent study showed that in addition to the Trp residues, the HDAg binding site on S-HBsAg also includes a tyrosine residue at position 200 [101]. Intrestingly, the Trp-rich motif in S-HBsAg is strictly conserved in all HBV genotypes as well as in WHV and WMHBV, suggesting that it plays an essential function in the HBV life cycle. However, this assumption was contradicted by the observation that an alanine substitution for Trp-196, -199 and -201 had no effect on the HBV replication cycle [102]. The reason for the Trp-rich motif conservation in all Orthohepadnaviruses may just be a consequence of the overlap between the envelope proteins and polymerase (Pol) genes, a characteristic feature of the HBV genetic organization and a consequence of the small size of the HBV genome. In fact the ORF of HBV Env is entirely included in that of Pol, and the DNA sequence surrounding Trp-196 codon in S-HBsAg ORF also encodes the Tyr-Met-Asp-Asp motif of the Pol catalytic domain in the minus-one reading frame. Since there is a strict requirement for a Tyr-Met-Asp-Asp motif in Pol, there is no other possibility than Trp at position 196 in S-HBsAg. By extrapolation, a few S-HBsAg residues (i.e., Trp-199, Tyr-200 and Trp-201), in addition to Trp-196, might also be solely conserved because they share a DNA coding sequence with the Pol catalytic domain. Thus a conserved and essential Tyr-Met-Asp-Asp motif in Pol imposes a conserved, Trp-rich motif in S-HBsAg, which is fortuitously used by HDV as a matrix domain for assembly. Note that this HDV matrix domain could represent a target of choice for anti HDV therapy, because a drug competing with HDV RNP for binding to S-HBsAg would not lead to the occurrence of HBV escape mutants.
Clearly, HBV appears as the best equipped virus to help HDV overcome its propagation defect, for the following reasons: (1) in HBV-infected cells , there is always a huge overproduction of envelope proteins that self-assemble into empty lipoprotein transport vesicles—in other words, a guaranteed, free and reliable cell export machinery, (2) the HBV envelope proteins are very flexible, in that they can assemble into various types of particles, namely the spherical SVPs (22 nm in diameter), the empty filaments (22 nm in diameter, up to several hundred nanometers in length), and the 42-nm HBV virions, (3) the small size of the HBV genome , which imposes the pol/env genes overlap at the origin of the HDV matrix domain conservation in S-HBsAg. Stoichiometry also appears in favor of S-HBsAg/RNP interaction since an average infected cell contains an estimate of 6 × 106 copies of HDAg proteins and approximately 105 copies of genomic HDV RNA [26]. Noteworthy, HBV and HDV envelopes may differ from each other with regard to the relative amount of L-HBsAg, estimated at up to 25 % of the total surface proteins for HBV [103] and only 5 % or less for HDV [2, 104].
HDV Entry
Since HDV and HBV virions are coated with the same surface proteins, they are expected to use identical host factors for cell surface attachment and receptor binding. Based on this assumption, the HDV model has been used to study the HBV envelope protein function at viral entry. In fact, in vitro HDV infection assays offered several practical advantages: (1) HDV entry into susceptible cells leads to very high levels of replicating HDV RNA (up to 300,000 copies per cell) that is easily detectable by Northern blot hybridization as early as 6 days post inoculation, and (2) infections being nonproductive in the absence of the helper HBV, the level of intracellular viral RNA that accumulates in an infected cell is directly proportional to the viral titer of the inoculum [105]. These characteristics have been crucial to the recent identification of sodium taurocholate cotransporting polypeptide (NTCP) as a common HBV/HDV receptor [106].
The initial attachment of HBV/HDV particles is mediated by cell surface heparan sulfate proteoglycans [107–110]. Glycosaminoglycan (GAG) side chains of proteoglycans are used by various viruses as primary docking sites, and they are clearly implicated in HBV and HDV entry. HBV and HDV virions were shown to bind to GAGs at the surface of susceptible HepaRG cells, or to immobilized heparin. Furthermore, infection could be blocked by treatment of virions with heparin (or highly sulfated dextran sulfate), or upon treatment of HepaRG cells with heparinase prior to inoculation. The requirement for cell-surface GAGs as low-affinity receptor for HBV has also been demonstrated in primary cultures of Tupaia hepatocytes [108].
A ligand to cell-surface GAGs has been identified in the surface-exposed antigenic loop (AGL) , of the HBV envelope proteins [111]. This infectivity determinant was shown to depend upon cysteine residues involved in structuring the AGL-associated a-determinant [111]. The a-determinant is a conserved immune-dominant determinant bearing most of the HBV neutralizing epitopes. The AGL-associated infectivity determinant was precisely mapped to a set of conserved residues—cysteines and non-cysteines—predicted to cluster together in a network of disulfide bridges that underlies the a-determinant [112]. The HBV envelope proteins would mediate electrostatic interactions with negatively charged GAGs through the positively charged residues R122 and K141 within the AGL. In addition, there might be a participation of the pre-S domain of L-HBsAg in HBV virion binding to GAGs because the pre-S domain of L-HBsAg is positively charged and, as compared to the HDV envelope, the HBV virion envelope is enriched in L-HBsAg [109]. Although the AGL determinant is clearly essential for HBV/HDV entry, it is still unclear to what extent its binding to GAGs at the surface of human hepatocytes participates to species specificity and tissue tropism.
After the reversible and low affinity attachment of HDV/HBV virions to host cell surface GAGs, the pre-S1 domain of L-HBsAg binds with high affinity to a specific receptor. This receptor has been identified recently as NTCP, a bile acid transporter highly expressed at the basolateral membrane of differentiated hepatocytes [106, 113]. The critical role of pre-S1 in infectivity was first demonstrated using HDV particles [15]. Then numerous studies based on neutralizing antibodies and mutagenesis approaches, demonstrated that both HBV and HDV were dependent on pre-S1 for infectivity. This was also demonstrated using pre-S1 specific peptides in in vitro infection assays. These experiments clearly established that the integrity of the N-terminal 75 amino acid residues of pre-S1, including N-terminal acylation with myristic acid, was essential. Furthermore, a synthetic myristoylated pre-S1 peptide (Myr-pre-S1) encompassing residues 2–48 could block infection [114–121]. This lipopeptide has since been used extensively to characterize the early steps of HBV/HDV infection, and it was also instrumental in the identification of NTCP as a receptor [106, 118, 122, 123]. It has been proven a potent HBV/HDV entry inhibitor, and it constitutes a promising antiviral drug (Myrcludex-B) currently in clinical trials. In tissue culture, Myr-pre-S1 blocks HBV/HDV infection at nanomolar concentrations, and it is active upon a short preincubation of cells with the lipopeptide, suggesting its rapid and efficient targeting to the NTCP receptor at the hepatocyte surface [118].
Because of their identical envelopes, HBV and HDV have in common the very early steps of binding and internalization, but once released in the cytoplasm, the HDV RNP and the HBV nucleocapsid most likely segregate in separate pathways to reach the nucleus [124]. In support of an endocytic route for HBV entry, a recent study demonstrated that extraction of cholesterol from HBV virions reduced infectivity without affecting particle integrity, antigenicity and the ability to bind hepatocytes [125]. The cholesterol content of the viral envelope was dispensable for viral binding, but likely involved at a post-binding step in the entry process [125]. HBV/HDV internalization is thought to be mediated by endocytosis, but there is actually no consensus on the exact pathway. Using different in vitro infection assays, HBV entry has been reported to use caveolae, clathrin or macropinocytosis [126–128]. Therefore the question of the entry pathway(s) for HBV or HDV remains unresolved.
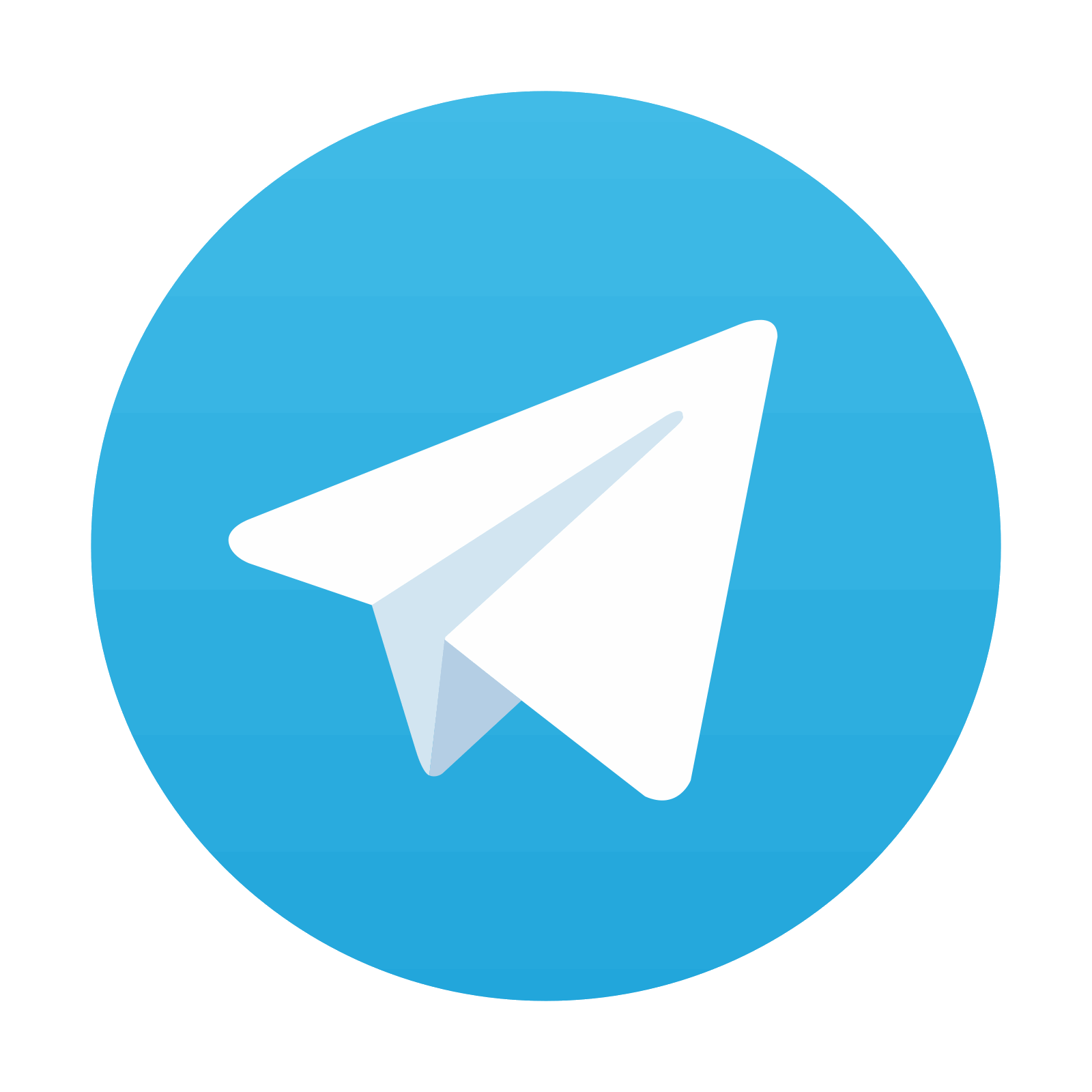
Stay updated, free articles. Join our Telegram channel
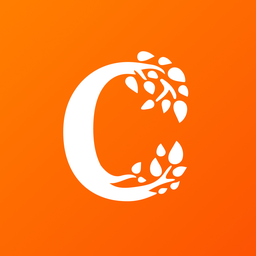
Full access? Get Clinical Tree
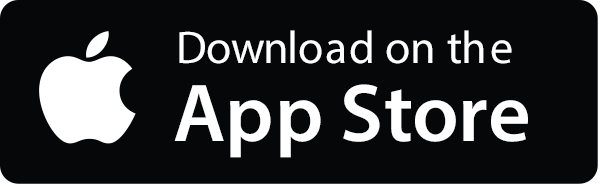
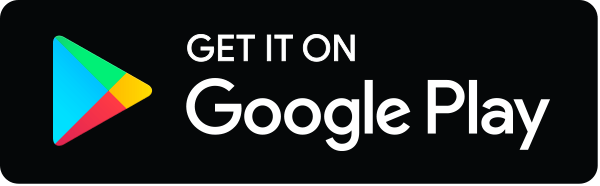