Abstract
The intestinal tract performs one of the most basic biologic functions, namely the harvesting of nutrients from ingested food. Moreover, the intestine participates broadly in numerous aspects of metabolism including regulating energy balance by influencing both energy intake and expenditure. While the mammalian intestine has an array of complex processes both to mediate efficient production of energy and key building blocks from ingested food and mechanisms to regulate how such materials are acquired and utilized, such systems are not operated exclusively in mammalian cells and enzymes. Rather, it is now appreciated that most, if not all, metabolic processes in the intestine are mediated by and/or greatly influenced by the gut microbiota, which is the collective term for the microorganisms that inhabit the gastrointestinal tract. In particular, the approximately 100 trillion bacteria that inhabit the human intestine are now well appreciated to directly participate in the digestion of ingested food and, moreover, produce an array of mediators that can have broad impacts on an array of metabolic processes. This chapter reviews some of the ways that gut bacteria impact metabolism.
Keywords
Carbohydrate degradation, Energy harvest, Micronutrients, Tryptophan, Bile acids, Low-grade inflammation, Insulin resistance, Satiety signaling, Gut-brain axis, Lipopolysaccharide, Flagellin, Mucus, IL-22
35.1
Introduction: Microbiota: The Intestine’s Best Frenemy Forever
The mammalian intestine is inhabited by a diverse collection of microorganisms collectively referred to as the gut microbiota. While the accuracy of the often-cited estimate that the human gut contains about 100 trillion bacteria has recently been questioned, in any case, there are certainly a lot of bacteria present and there is strong evidence that they impact numerous aspects of the host phenotype. Estimates of the numbers of bacterial species present in individual hosts can vary from a few hundred to upwards of 10,000 in part depending upon the specific methodologies (primarily DNA sequencing) and biological definitions of species. In any case, there is broad agreement that, in terms of numbers of bacteria, the human gut is dominated by 2 anaerobic Gram-positive phyla, namely Bacteroidetes and Firmicutes . Thus, logically, much of the study of how gut bacteria contribute to metabolism has focused on species within these phyla. The next most abundant phyla is Proteobacteria , which includes facultative anaerobes, some of which are considered “pathobionts,” a term which indicates to promote, but not necessarily cause, chronic inflammatory disease. The next most abundant phyla, at least based on analysis of human stool samples, is Actinobacteria , which also plays a role in metabolism. While the microbiota also contains fungi and viruses that may also contribute to metabolism, their roles are not well studied and would not be discussed here.
While core phyla are generally similar among humans, there are clear differences in relative abundance of these phyla. Moreover, there are clear differences in species composition. While species compositions of individuals can change somewhat over time and be impacted by various disease states (e.g., inflammation) and changes in diet, overall, the changes over time within an individual are much smaller than the differences between individuals. Hence, the microbiota to which one is exposed at an early age is thought to have a life-long impact upon the composition of an individual’s microbiota throughout their lifetime. Hence, microbiota composition, at least in mice and likely in humans, is not only a distinct “finger print” of an individual but a nongenetic, that is, environmental, factor that influences phenotype. Indeed, as discussed herein, the specific composition of the microbiota will greatly influence how it impacts metabolism under various conditions. In general, when viewed holistically, the gut microbiota clearly has an overall benefit to the host in that germfree mice, which lack a microbiota, have considerable immune and metabolic defects with the latter resulting in a requirement for greater caloric consumption relative to body mass. However, that multiple mouse models of inflammation require a gut microbiota and that the composition of the microbiota is a determinant of disease indicate that the microbiota can also constitute a major threat to its host. In light of the benefits the microbiota confers on key areas of host biology and its potential to harm its host if not managed properly, and that early life composition has long-lasting effects, we refer to the gut microbiota as the hosťs best frenemy forever. Yet, the extent to which microbiota beneficially and/or detrimentally impacts host metabolism depends upon an array of factors including, but not limited to, diet and microbiota composition. This chapter will discuss some of the myriad of potential ways by which microbiota impact metabolism. While many of the findings discussed were generated from animal and in vitro models, we have tried to generally relate the results to human physiology.
35.2
Direct Impact of Microbiota on Ingested Food
The most basic way that the microbiota participates in metabolism is aiding in the breakdown of ingested macromolecules. In particular, the microbiota’s collective genome, referred to as the microbiome, encodes many more degradative enzymes than the hosts they inhabit thus enabling greater breakdown, and consequently energy harvest from a greater variety of food sources. While this is most obviously true in ruminant animals, the concepts are now generally viewed to be widely applicable to other mammals albeit clearly to a lesser extent. While energy harvest is a crucially important outcome from such interactions, microbiota’s role in digestion also involves the generation of a range of metabolites.
35.2.1
Carbohydrate Degradation
Mammalian hosts lack many enzymes needed to digest complex carbohydrates present in many of the plants they ingest. Hence, ruminants are largely, if not absolutely, dependent upon gut bacteria to breakdown and ferment ingested complex polysaccharides. While other herbivores and omnivores are less reliant on microbial fermentation, it has nonetheless been estimated that 10% of the dietary energy intake of humans is dependent upon gut bacteria. Breakdown of carbohydrates, including glycoproteins, oligosaccharides, and polysaccharides, is mediated by a group of enzymes collectively referred to as carbohydrate-active enzymes (CAZymes). The chemistry of CAZymes is a highly complex area whose understanding continues to evolve rapidly and thus can best be monitored by the related web sites cazy.org and cazypedia.org , which are maintained by a collective of carbohydrate chemists. Classes of CAZymes include glycoside hydrolases, which hydrolyze or rearrange glyosidic bonds, polysaccharide lysases, which catalyze the nonhydrolytic cleavage of glyosidic bonds, and carbohydrates esterases, which catalyze the deacylation of saccharides ( Fig. 35.1 ). As of 2017, there are 145 families of glycoside hydrolases, 27 families of polysaccharide lysases, and 16 families of carbohydrates esterases. For each of these families, hundreds of distinct members have been identified in bacteria and comparatively few in eukaryotes. They are also important in the digestion of carbohydrate binding molecules (CBM), which play a diverse role in the sequestration of these molecules and are presumed to aid in their breakdown. The CAZY database lists over 100,000 identified CBM classified into 81 families. Analogous to CAzymes, most known CBM are of bacterial origin.

Identification of CAZ and CBM has largely relied on sequence-based metagenomics approaches, which make it very difficult to assign roles of specific molecules in carbohydrate breakdown; but it is presumed to be a highly collaborative process involving many enzymes in many bacterial species whereas individual bacteria lack the wherewithal to efficiently metabolize many individual complex saccharides. Such interactions are best understood in the rumen wherein, for example, degradation of the plant cell wall component xylan requires five distinct enzymatic activities suggested to be provided by a range of Ruminococcus and Prevotella species. Microbiota also play a central role in the degradation of complex carbohydrates in humans. While simple carbohydates are readily broken down in the upper GI tract, approximately 20–60 g of ingested carbohydrates are thought to have reached the colon. Such carbohydrate includes resistant starch, which consists largely of plant-derived oligosaccharides. Enzymes and bacteria involved in the metabolism of resistant starch in the human intestine are not well defined but, nonetheless, such resistant starches are fermented in the colon and give rise to beneficial molecules discussed below. The breakdown of plant cell wall components such as cellulose in the human gut is even less well understood. Briefly, humans lack enzymes needed to degrade cellulose but yet are able to breakdown this complex carbohydrate. For example, a study wherein participants were adapted to a cellulose-enriched diet found that about 70% of cellulose was degraded as it passed through the GI tract although this result is variable in terms of both individuals studied and the specific type of fiber utilized. In any case, bacteria with the capacity to degrade cellulose have been isolated from the human intestine. Such bacteria were classified as Ruminococcus , Clostridia , and unknown species.
In contrast to cellulose, a range of dietary fibers are readily fermented by gut bacteria and are, accordingly referred to as fermentable or soluble fibers. Such readily fermentable fibers include fructans, galactooligosaccharides (GOS), and fructooligosaccharides (FOS). These fibers are efficiently metabolized by a range of gut bacterial species whose abundance is, consequently, increased upon the consumption of such fibers. Such changes in microbiota composition, and how it impacts metabolism, will be discussed below. Here, in terms of the role of the microbiota in breaking down such products, it is clear from mouse studies that, in the absence of a microbiota, fibers such as inulin that are efficiently fermented in conventional mice are not degraded in germfree mice. The breakdown of such fibers requires a range of distinct glycosidase activities. GOS are especially abundant in human breast milk and accordingly, a range of CAZ needed to degrade GOS are expressed by a range of Bifidobacterium species. Specifically, Bifidobacteria produce multiple glycosidases, transporters, and metabolic enzymes that enable it to use an unusually wide variety of carbohydrates. Collectively, these proteins comprise a central fermentative pathway referred to as the “Bifid shunt,” which is presumed to give Bifidobaterium a great growth advantage in a GOS-enriched diet and may produce a range of molecules that benefit the host.
35.2.2
Role of Microbial-Mediated Carbohydrate Breakdown in Human Metabolism
The general importance of microbial-mediated carbohydrate breakdown in nonruminant mammals can be appreciated by the aforementioned estimate that it accounts for 10% of total caloric intake in humans. The impact of such a difference can be seen in the pioneering work of Jeff Gordon and colleagues, who compared conventional and germfree mice, which were fed the same autoclaved chow. Germfree mice ate over 35% more chow to achieve similar total body weights but yet stored less energy in that they had a 40% reduction in fat mass. Germfree mice also exhibit a near-complete loss of colonic short-chain fatty acids (SCFAs), which are an efficiently used energy source for mammalian hosts, and an increase in unharvested calories that remained in the feces of germfree mice. Together, these results strongly suggest that complete absence of a microbiota reduces efficiency of energy harvest in a manner that contributes to altered state of energy balance characterized by leanness despite high levels of food consumption. The extension of this logic to the less extreme case of a host that has a microbiota has led Gordon and colleagues to postulate that not all microbiotas are equal in their capacity to harvest energy but rather that some microbiotas do a better job of breaking down complex carbohydrates that are available to them and hence microbiota composition is a determinant of energy intake in general and, consequently, a risk factor of adiposity in particular. Evidence that supports this notion includes that both obese mice, including mice that are obese due to impaired satiety signaling, namely leptin-deficient mice (Ob/Ob) and obese humans have alterations in gut microbiota that are associated with the obese state, which is itself associated with higher levels of cecal SCFA and lower levels of calories in feces indicating that the microbiotas of the obese hosts are indeed associated with increased energy harvest. Furthermore, transfers of such microbiotas from obese humans to germfree mice transferred increased microbial fermentation, as read out by SCFA levels relative to germfree mice conventionalized with microbiotas from lean hosts. Furthermore, germfree mice receiving microbiotas from obese mice displayed reduced levels of energy in their feces, as assayed by bomb calorimetry, indicating they had more completely harvested the potential energy available in food they had ingested. Lastly, transplant of such microbiotas with “increased capacity for energy harvest” resulted in increased adiposity in mice receiving these microbiotas. Metagenomic analysis of feces both donors and recipients of such microbiotas indicate that such increased energy harvest correlates with a microbiota enriched in bacterial genes-related breakdown of complex carbohydrates.
The notion that microbiota composition is a determinant of energy harvest is supported by agricultural practices which find that feed efficiency, in this case, defined as total saleable meat/feed cost is enhanced by administering low-dose antibiotics although it is not clear if this increases energy harvest per se—this question is more difficult to address in large farm animals wherein many potentially lost calories are emitted in gasses such as methane—or might reflect that the antibiotics make the animals grow faster, thus reducing calories used to sustain life.
Another observation in humans that supports the importance of microbiota composition as a determinant of energy harvest is a study from Walker and colleagues, who observed that, among a group of healthy volunteers there was heterogeneity in microbiota composition that correlated with the ability to ferment dietary resistant starches. Specifically, while most individuals fermented over 96% of administered resistant starch, some donors, particularly ones who harbored very few Ruminococci species had over 60% of the resistant starch pass through their GI tracts unfermented. Together, these observations highlight the potential of the microbiota to impact metabolism by energy harvest. Yet, this is a complex area wherein some mouse studies have found microbiota not to be necessary for diet-induced obesity, while others have found it is strain dependent. Moreover, some human studies, including ones from Gordon’s group, report that more efficient metabolism of carbohydrates to SCFAs associates with a lean state. In any case, as will be discussed below, there are a number of other ways the microbiota can also impact metabolism and the relative importance of these different mechanisms in humans, in most situations, is not yet clear.
35.2.3
Role of Microbiota in the Breakdown of Proteins
The role of the microbiota in the metabolism of proteins has been studied much less extensively than that of carbohydrates although both nutrient types are largely catabolized simultaneously by host and microbial enzymes. In contrast to the case of carbohydrates wherein mammals, without help from bacteria, lack the wherewithal to degrade many complex polysaccharides, both bacteria and mammals are capable of catabolizing most proteins. Hence, the relative contributions of bacterial versus mammalian proteases in hydrolyzing proteins to amino acids and small peptides are not clear. In any case, a significant portion, perhaps 10%–20% of the amino acids derived from ingested proteins is thought to be initially be taken up by bacteria in the small intestine although tracer-based studies used to reach these estimates are prone to a wide variation. Amino acids taken up by bacteria can be used for anabolic purposes (largely protein synthesis) or catabolism to make various structural molecules (amines, polyamines) or deaminated to make ketoacids and various lipids, which are primarily used by the bacteria themselves ( Fig. 35.2 ). Additionally, bacteria are capable of de novo synthesis of most (to some extent) all amino acids and labeling studies have shown, for example, that a significant portion of nitrogen from ammonium chloride administered to mice ended up in host proteins in conventional but not germfree mice. Thus, while the net consequence of much of bacterial metabolism of proteins would seem to be influenced by the availability of amino acids and small peptides to the host although the extent to which is actually the case is not well defined. In any case, a number of products of amino acid breakdown are released by bacteria that have a range of impacts on host metabolism.

Some deaminated amino acids are ultimately released as products used by enterocytes primarily for purposes of supplying energy such as short-chain and branched-chain fatty acids. Indeed, these are preferred fuels of enterocytes. Moreover, four other classes of compounds present in the colon are largely the consequence of bacterial metabolism, namely ammonia, hydrogen sulfide, polyamines, and indols/phenols. Ammonia is abundant in the colon in feces in most animals and there is strong evidence that the source is gut bacterial catabolism of peptides. Levels of ammonium, parallel concentration of bacteria increase with increased protein consumption and are greatly reduced by depletion of gut bacteria by antibiotics or germfree approaches. Indeed, the lack of fecal ammonium is the primary reason cages of germfree mice can be changed much less frequently than conventional mice. Ammonia is produced via urease acting on urea, which is resulting from the deamination of amino acids. While urease from Helicobacter pylori is the best-studied form of this enzyme, numerous other bacteria, including species common in the colon are able to produce ammonia suggesting these bacteria possess this enzyme. While ammonia can be taken up by enterocytes, and can be used to make glutamine, ammonia is generally thought to interfere with an array of basic intracellular metabolic processes and hence requires detoxification or excretion. Another intestinal gas produced by gut bacteria is hydrogen sulfide (H 2 S). H 2 S can be produced from sulfur-containing amino acids by fermentation by a well-defined set of chemical reactions. Production of H 2 S from proteins can be mediated by a range of common gut bacteria including Clostridia and Enterobacter species. While there are a number of other routes for bacteria to produce H 2 S, that elevation of protein consumption increases H 2 S levels argues that breakdown of proteins play a significant role in vivo. Like ammonia, H 2 S is considered a metabolic troublemaker. Hence, there are number of means by which the host sequesters and detoxifies H 2 S but, nonetheless high levels of both H 2 S and ammonia have been proposed to contribute to a range of disease states. Polyamines can be produced from decarboxylated amino acids by a range of bacteria, although synthetic pathways have primarily been studied in Escherichia coli. While human cells also produce a range of polyamines, some polyamines, for example, cadaverine, are microbial specific and accordingly, levels are eliminated by antibiotic treatment. While polyamines are increasingly appreciated to play an important role in a number of processes related to host-microbial interactions, including the formation of biofilms, the extent to which bacterial-produced polyamines play essential or detrimental roles in host metabolism is not well defined.
One of the starkest means by which microbiota metabolism of ingested food results in production of metabolites whose generation depends upon the presence of the microbiota is via acting upon aromatic amino acids. Specifically, studies by Smith and McFarlane demonstrated that a range of anaerobic bacteria present in the colon, including Bacteroides , Lactobacillus , Bifidobacterium , and Clostridia species, can ferment aromatic amino acids. As outlined in Fig. 35.3 , tyrosine is a range of compounds to end up as phenol, ethylphenol, propylphenol, and especially p-cresol, which is likely the major end product of tyrosine metabolism by bacteria in vitro and in vivo. Phenylalanine is metabolized to phenylacetate and phenylproprionate. Tryptophan is metabolized to a range of indols including indole, indolpyruvate, tryptamine, indolproprionate, and skatole. Many of these molecules are taken up by the host and can be found in blood and urine. Indeed, untargeted metabolomics studies of blood and urine generally find bacterial metabolites of aromatic amino acids to be the most enriched products in conventional mice relative to germfree animals. Specifically, a number of phenyl derivatives, including phenylsulfate, p-cresol sulfate, and phenylproprionylglycine, were readily detected in serum from conventional mice but were undetectable in germfree mice while levels of serum hippuric acid were 17-fold higher in conventional mice. Indolproprionic acids (IPA) were also found to be present only in conventional mice while serotonin, which can be derived from IPA, was 2.8-fold higher in conventional mice. Conversely, tryptophan itself was about twofold higher in germfree mice suggesting that lack of its metabolism in the absence of a microbiota increases the pool of this product for downstream metabolism by host pathways. Overall, while the relative roles of the most indol/phenolic compounds are not well understood that microbiota-derived phenols, especially hippuric acids and cresols, are excreted in urine argues that the host deems these bacterial-generated metabolites as somewhat toxic. Hence, much of the current research related to these molecules has focused on developing metabolomics based approaches to define metabolic signatures of microbial impacts upon health and disease. In accord with the general notion that altered microbiota compositions influence such outputs, transfer of distinct microbiotas to germfree mice correlated with clear changes in levels of these phenolic compounds in the host. Indols are increasing appreciated to play central roles in both bacterial-bacterial and bacterial-host communications in general and in maintaining a stable host-microbiota relationship in particular, at least in part by activating the aryl hydrocarbon receptor to drive a variety of immunomodulatory effects. A lot of further work is needed to understand how bacterial-derived indols influence host metabolism and how differences in bacterial populations in the gut influence the production of such metabolites. In any case, microbiota metabolism of aromatic amino acids seems likely to be one major means by which direct microbial action on ingested proteins broadly influences host metabolism.

35.2.4
Lipids
Numerous gut bacteria are capable of using a variety of lipids as a primary carbon source. Yet, the extent to which gut bacteria might directly take up and metabolize dietary lipids has been a subject of much research. Rather, gut bacteria are known to have an indirect but major impact on lipid metabolism via their modification of primary bile acids to secondary bile acids. Some of these effects involve lipid trafficking while others are mediated by localized and systemic receptor-mediated processes, which will be discussed below.
35.2.5
Micronutrients
The microbiota is also capable of metabolizing and generating a number of micronutrients. Briefly, both select bacterial species and in vitro suspensions of complex microbiota can produce a range of water-soluble vitamins including thiamine, riboflavin, niacin, biotin, and folate that play a role in a wide variety of metabolic processes ( Table 35.1 ). While high levels of folate are produced by a range of colonic bacteria and can be modulated by fermentable fiber intake, the extent to which there is a net production or consumption of other water-soluble vitamins is unclear. Microbiota can also synthesize a range of fat-soluble vitamins including vitamins A and K. In the case of vitamin A, a range of potential routes of synthesis in which bacterial enzymes might participate have been described but, again, the relative importance of such pathways is not well defined. In contrast, a key role for microbiota in generating vitamin K is documented. In particular, gut bacteria can generate Vitamin K 2 in accord with observation that Vitamin K 2 is found in fermented foods as well as in products (meat and milk) from ruminant animals. A range of bacteria, including Bacteroides, Enterobacter , and Veillonella , can make various subforms of K 2 , referred to as congeners. Given that plants are capable of direct Vitamin K 1 synthesis and that many foods contain K 2 (even if they lack live bacteria at the time of ingestion) raises the general question of the importance of one’s microbiota in Vitamin K metabolism. While overall dietary intake presumably is a major determinant in the extent to which individuals rely upon their microbiota to produce this key nutrient, Vitamin K deficiency is perhaps the best-documented micronutrient deficiency of germfree mice arguing that microbiota likely play an important role in producing Vitamin K in normal hosts on a standard diet. It is anticipated that future studies will reveal key roles for the microbiota in mediating other specific types of vitamin sufficiency and deficiency in health and disease.
Vitamin | Function | Absorption | Transport |
---|---|---|---|
Thiamine | Energy metabolism (sugar) and ATP formation | Absorption in the large intestine via sodium-dependent pH-sensitive carrier | The human thiamine transporters (hTHTR-1 and -2) are expressed in human colonocyte. Thiamine can enter the colonocytes in the free and phosphorylated forms and thus can contribute to the colonic energy metabolism. Whether thiamin is delivered to the systemic circulation is unknown |
Riboflavin | Potential redox system. Cofactor for folate and vitamin B 6 activation | Uptake is adaptively regulated by the concentration of riboflavin in the diet | Riboflavin transporters (RFVT-1, -2, -3) are present in the colonocytes and mediate the uptake of riboflavin in colonocytes and intestinal cells. No data are available regarding influence on systemic status |
Niacin | Energy metabolism, redox system | Uptake into colonocytes is regulated by extracellular substrate availability | High-affinity transporter; important for niacin concentration in colonocytes. No data are available regarding influence on systemic status |
Biotin | Immune function, substrate metabolism | Colonocytes absorb biotin via a carrier-mediated process | Transport via sodium-dependent multivitamin transporter (SMVT). No data are available regarding influence on systemic status |
Pantothenic acid | Energy metabolism | Colonocytes absorb pantothenic acid via a carrier-mediated process | Transport via SMVT |
Folate | Methyl donor, nucleotide synthesis | Colonocytes absorb folate via a carrier-mediated process | Transport via reduced folate carrier and proton-coupled folate carrier. Evidence for basolateral absorption and contribution to systemic status |
35.3
Modulation of Metabolism by Microbiota-Generated Products
In addition to directly participating in the digestion of ingested food, there are a myriad of means by which microbiota influence host metabolism. Some of these means are discussed here in the general order of their proximity to digestion and energy harvest.
35.3.1
Bile Acids
35.3.1.1
Modification of Bile Acids by Microbiota: Impact Upon Metabolism
Bile acids are produced from cholesterol in the liver and secreted via the gall bladder into the duodenum, from where they will flow down the intestinal lumen where they will be largely re-absorbed, alone or attached to lipid particles, returned to the liver, which is a circuit collectively referred to as the enterohepatic circulation. Such bile acid cycling has long been appreciated to play an essential role in emulsifying ingested lipids, thus both protecting the host from direct effects of these molecules (which can themselves have dangerous detergent-like properties) and, moreover mediating their uptake and subsequent transport and metabolism. Furthermore, bile acids are now well appreciated to activate both nuclear receptors and cell surface receptors that have a broad range of metabolic effects. While bile acids share key structural features, particularly their hydrophobic backbone and polar acidic group, there are numerous species of bile acids that vary in their hydrophobicity, and thus the ability to traffic lipids and in their ability to activate or antagonize receptors. While some of the structural variations are produced in the liver, in that the humans make about a half dozen primary, the microbiota has a dramatic effect on the overall bile acid profile, which results in broad effects on metabolism.
35.3.1.2
Modification of Bile Acids by Gut Microbiota
Bile acids are saturated, hydroxylated C24 cyclopentanephenanthrene sterols. Primary bile acids, such as cholic and chenodeoxycholic acids, are synthesized from cholesterol in the liver, conjugated to either taurine or glycine, and then excreted through the canaliculi to the biliary system. Following their excretion to the gastrointestinal tract, a portion of bile acids will be deconjugated and modified by bacterial metabolism, including oxidation, esterification, desulfatation, and epimerization, ultimately leading to the presence of over 50 different secondary bile acids in human feces, with subsequent minor modifications leading to numerous species of tertiary bile acid species ( Fig. 35.4 ). The initial step in the production of secondary bile acids is to deconjugate them, which is mediated by bile salt hydrolase (BSH). All gut bacteria are thought to encode at least 1 BSH and many bacteria encode several leading to speculation that the ability to deconjugate bile acids is an essential fitness factor for gut bacteria due to primary bile acids being dangerous to bacteria, potentially destroying their membranes and interfering with various bacterial metabolic processes. Deconjugation of the polar conjugates makes bile acids more hydrophobic and, consequently, less dangerous to bacteria. Such increased hydrophobicity results in reduced re-absorption of bile acids and rather favors their loss on feces. Hence, germfree mice exhibit a complete loss of secondary bile acids resulting in increased levels of primary bile acids in liver and a stark reduction of bile acids in colon and feces, because primary bile acids are reabsorbed so efficiently. In the normal case wherein microbiota is present, deconjugated bile acids, some of which will escape enterohepatic recirculation, will be modified by bacteria in the lower GI tract. A range of modifications are possible including oxidation-reduction, amidation, esterification, and sulfation to result in a diverse repertoire of secondary with some analytic schemes reporting the quantitation of upwards of 50 bile acids. While the specific microbial enzymes that mediate such modifications have not been well defined, although some have been identified, overall, they appear to exhibit a high degree of variance among bile acid profiles that are very much impacted by microbiota composition. Conversely, bile acids clearly impact microbiota composition in that direct addition of bile acids to the gut and/or pharmacologic interference alters microbiota composition. Hence, the relationship between bile acids and microbiota composition is best thought of as being one of dynamic equilibrium. In any case, microbiota composition has a clear effect on bile acid levels, which in turn has a myriad of ways of impacting metabolism.
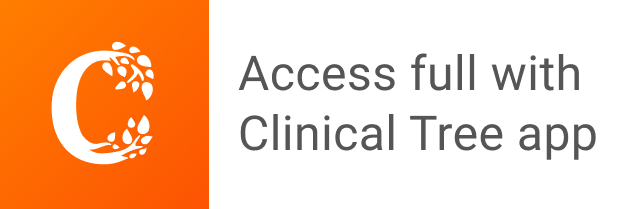