Abstract
Intestinal lipid transport is coordinated through pathways that facilitate uptake and metabolic channeling of fatty acids, monoglycerides, lipovitamins, and sterols into complex lipid for temporary storage and systemic delivery. The key rate limiting steps include transport across the brush-border membrane, vectorial delivery within apical cytoplasmic compartments to the endoplasmic reticulum (ER), and initiation of lipoprotein biogenesis. Within the ER, primordial lipid droplet particles are remodeled and undergo maturation through the Golgi profiles and nascent intracellular chylomicrons are eventually secreted into the pericellular basolateral spaces and lymphatic fenestrae. We review current understanding of intestinal lipoprotein biogenesis and the role of dominant and modifier genes in coordinating chylomicron assembly and secretion. We further discuss developments in understanding how intestinal lipid (including fatty acid and cholesterol) transport is disrupted in selected genetic disorders, with new insights into critical pathways, their physiologic regulation, and potential utility as pharmacologic targets.
Keywords
Apolipoprotein B, Microsomal triglyceride transfer protein, Genetic defects, Fatty acid transport, Cholesterol transport, Chylomicron formation, High-density lipoprotein, ABC transporters, Lipid droplets
49.1
Major Pathways and Genes Involved in Intestinal Lipid Droplet Formation and Triglyceride-Rich Lipoprotein Assembly
In summary overview, long-chain fatty acids (LCFAs), whether monomeric or in the form of monoglycerides, represent the major substrate for the generation of triglyceride- rich lipid droplets (LDs) and in turn for the assembly of triglyceride-rich lipoproteins. Apical uptake of LCFAs occurs predominantly in the proximal small intestine (duodenum, jejunum), although the ileum has the capacity to absorb LCFA and expresses all the requisite fatty acid and cholesterol transporter genes. Uptake across the intestinal brush border is facilitated by micellar solubilization of LCFA, which promotes their access to the apical surface and microvillus membrane. Diffusion across the microvillus membrane is both passive (i.e., along a concentration gradient) and also at least in part carrier mediated ( Fig. 49.1 ). Among the apical membrane proteins implicated in LCFA uptake are the multiligand transporter CD36 and the multifunctional glycoprotein milk fat globule-epidermal growth factor 8 (Mfge8). Earlier work implicated a role for CD36 in intestinal LCFA uptake. The impact of this pathway in humans, however, has yet to be established. More recently, new insight into the mechanisms involved in CD36-mediated regulation of apical lipid transport has emerged from findings linking a dynamic signaling pathway in which Mfge8 coordinates LCFA uptake through pathways including integrin-dependent phosphorylation of key intermediates (Akt and mTOR), which in turn promote translocation of CD36 from intracellular vesicular pools back to the apical membrane ( Fig. 49.1 ). This apical membrane distribution of CD36 is proposed to promote uptake of LCFA, which are then reassembled into triglyceride-rich LDs in the apical pole of the enterocyte as a temporary storage pool destined for chylomicron production ( Fig. 49.1 ). Further work has shown that Mfge8 also plays a key role in the mobilization of these LDs by promoting hydrolysis upon interacting with key heterodimeric integrins ( Fig. 49.1 ), which then promote triglyceride hydrolase activity. Other LD-associated proteins have been implicated in regulating postprandial lipid absorption, including comparative gene identification-58 (CGI-58), where genetic ablation led to accumulation of large apical LDs and impaired intestinal lipid delivery into the systemic circulation. These new findings together point to a revised model of intestinal lipid absorption in which cytoplasmic LDs represent a metabolically dynamic organelle from which lipid substrates are mobilized for chylomicron formation as well as participating in other metabolic processes (reviewed in Ref. ).

Once dietary lipids (LCFA, sterols, and fat-soluble vitamins) have been transferred into apical domains of small intestinal enterocytes, the process of their vectorial delivery into the systemic circulation via the intestinal lymph is coordinated through the assembly of large, vesicular particles called chylomicrons. A similar but not identical process occurs in the liver, where endogenous and exogenously derived lipids are assembled into slightly smaller particles called very low-density lipoproteins (VLDLs). Two dominant genes regulate the initiation of intestinal chylomicron assembly, specifically apolipoprotein B (apoB) and microsomal triglyceride transfer protein (MTTP). ApoB is the structural protein surrounding nascent intestinal lipoproteins, which are formed following the transfer of bulk membrane lipids mediated by MTTP. Details of the structure, function, and genetic regulation of these two genes are discussed below.
49.1.1
ApoB: Protein Structure and Functional Domains
ApoB is a structurally unique, obligate integral component of the surface of VLDL and chylomicrons. The APOB gene is located on the short arm of human chromosome 2 and in the syntenic locus of mouse chromosome 12. The liver and small intestine represent the major sites for apoB gene expression, although apoB is also expressed at low levels by human placenta, cardiomyocytes, and kidney proximal tubules. The full-length apoB protein (ApoB100) is a (Mr 550 kDa) hydrophobic polypeptide composed of 4536 amino acid residues. Human apoB100 contains three amphipathic α-helical domains alternating with two β-strand domains in an NH 2 -α 1 -β 1 -α 2 -β 2 -α 3 -COOH configuration. A truncated form of apoB (ApoB48) is generated in the small intestine through posttranscriptional RNA editing (described below) in which the translated apoB48 protein comprises the first two domains (α 1 -β 1 -) and a portion of the third domain (α 2 ). This structural configuration is organized with a single copy of apoB48 on each lipoprotein particle. The β 1 and β 2 domains likely exist in the form of belt-like structures wrapped around the particle and are predicted to be irreversibly associated with the lipid core of the lipoprotein, whereas the α-helices of the α-domains, which are similar to those found in the “exchangeable” apolipoproteins such as apoAI and apoE, are thought to confer reversible lipid-binding properties. The α 1 domain is a highly disulfide-bonded globular domain, containing 7 of the 8 paired disulfide bonds found in apoB100. Most of the plasma apoB-containing particles are metabolized via the low-density lipoprotein (LDL) receptor-mediated pathway after they are secreted into the blood stream. The LDL receptor-recognition sites for apoB reside within the β 2 domain of apoB100 and are thus absent in apoB48. As a result, uptake of apoB48-containing particles (chylomicron remnants) is mediated by the interaction of apoE with the LDL receptor-related protein 1 and other lipoprotein receptors.
49.1.2
Apolipoprotein B mRNA Editing: Overview, Molecular Mechanisms, and Functional Relevance
As noted above, mammalian small intestine synthesizes a truncated species of apoB, referred to as apoB48. The underlying mechanism of apoB48 production is tissue-specific posttranscriptional C-to-U RNA editing of the primary transcript that introduces a translational stop codon. The net result is that intestinal chylomicron assembly, and triglyceride secretion is coordinated through apoB48 production while hepatic VLDL assembly and secretion is coordinated through apoB100 production. The functional impact and presumed survival advantages of apoB48 production are discussed below.
49.1.2.1
ApoB mRNA Editing: Molecular Mechanism and Tissue-Specific Regulation
New insights have recently emerged into the molecular machinery required for intestinal apoB C-to-U RNA editing. The minimal requisite components for C-to-U RNA editing include Apobec-1 and one or more RNA-binding proteins, which together promote site-specific cytosine deamination in selected RNA targets. Apobec-1, the catalytic deaminase, is required for C-to-U RNA editing because its genetic deletion in mice eliminates C-to-U RNA editing of intestinal apoB as well as eliminating C-to-U RNA editing of all other targets. Moreover, the conditional rescue of Apobec-1 in Apobec-1 intestine-only transgenic mice restores C-to-U RNA editing of apoB and the other known targets to physiological levels. However, while Apobec-1 is essential for C-to-U RNA editing both in vivo and in vitro, apobec-1 alone is not sufficient. C-to-U editing of apoB RNA requires an auxiliary RNA-binding cofactor, Apobec-1 complementation factor, ACF. ACF was identified as the requisite auxiliary factor for Apobec-1-dependent C-to-U RNA editing of apoB in 2000 by two groups, based on its ability to promote in vitro RNA editing of a synthetic apoB RNA template. New information suggests that, while ACF indeed functions in vitro to promote site-specific RNA deaminase activity of Apobec-1, ACF appears completely dispensable to C-to-U RNA editing in vivo. Germline deletion of ACF in mice was early embryonic lethal (E 3.5 days), precluding attempts to discern a role in C-to-U RNA editing of apoB. However, a recently developed conditional ACF-knockout mouse (using a Cre driver active after E 6.5 days) yields viable offspring with no ACF in any tissue, yet with no change in C-to-U RNA editing of apoB or of any other Apobec-1-dependent target. Thus, while ACF binds apoB RNA and Apobec-1 and may be sufficient to mediate Apobec-1 deaminase activity on a synthetic RNA template in vitro, it is not required for Apobec-1 activity in vivo. Rather, another RNA-binding protein (RBM47) functionally related to ACF may be the dominant auxiliary factor for RNA editing. RBM47 was identified in a genetic screen for developmentally regulated genes involved in gut development in which RBM47-null mice exhibited neonatal lethality and stunted growth. In addition, RBM47-null mice exhibit decreased (although not zero) intestinal apoB RNA editing as well decreased C-to-U RNA editing of other Apobec-1-dependent RNA targets. Further studies demonstrated that recombinant RBM47 is sufficient to mediate Apobec-1-dependent deaminase activity on a synthetic apoB RNA template in vitro (similar to that shown for ACF). These data together suggest that RBM47 is both necessary and sufficient for Apobec-1-dependent C-to-U RNA editing, both in vitro and in vivo and strongly points to its role as a dominant cofactor for intestinal apoB RNA editing. More recent work has suggested a key role for RBM47 in supporting tissue growth, implying a broader function for this RNA-binding protein, beyond apoB RNA editing.
49.1.2.2
Functional Significance of apoB C-to-U RNA Editing
The tissue-specific distribution of apoB mRNA editing and its restriction in humans to intestinal apoB reflects the tissue and cell-specific distribution of Apobec-1, which is developmentally regulated and expressed in enterocytes and subepithelial cells throughout the luminal gastrointestinal tract. ACF expression, like that of RBM47, is found in almost all tissues in humans, rats and mice. Intestinal apoB mRNA editing is developmentally regulated, with a progressive increase in the proportion of intestinal apoB48 mRNA culminating in greater than 90% editing in postnatal mammalian enterocytes. These observations strongly suggest that RNA editing plays an important role in the developmental regulation of key pathways in intestinal lipid metabolism.
As alluded to above, many (more than 50) additional C-to-U RNA editing targets (beyond apoB RNA) of Apobec-1 have been identified, principally within 3′ untranslated regions of RNAs that contain the canonical apobec-1-binding site, an UUUN[A/U]U motif embedded within an A + U rich sequence. Accordingly, there is increasing interest in the possibility that both Apobec-1 and its RNA binding partners (ACF, RBM47) exhibit other important functions, beyond intestinal lipid metabolism. For example, crossing Apobec-1 −/− mice into Apc min /+ mice resulted in a dramatic attenuation of intestinal polyposis, suggesting that Apobec-1 may represent a genetic modifier of other dominant pathways, including those involved in intestinal malignancy. Other studies have demonstrated a role for ACF in modulating cytokine (specifically interleukin 6) mRNA stability and in the regulation of liver growth. More recent studies identified RBM47 as a selectable metastatic trait in breast cancer strongly supporting the possibility that Apobec-1, RBM47, and ACF each have a range of targets distinct from apoB mRNA, whose biological implications have yet to be clearly delineated.
49.1.3
MTTP: Functional Domains and Regulation
Initiation of apoB-containing lipoprotein formation ( Fig. 49.2 ) requires cotranslational lipidation of apoB via the lipid transfer function of MTTP. MTTP is neutral lipid transfer protein found in the ER lumen of apoB-producing cells, principally enterocytes and hepatocytes but also in cardiomyocytes, placenta, retinal, and proximal renal tubular epithelial cells as well as in cells that do not produce apoB, such as CD1-restricted T cells. MTTP exists as a 97 kDa protein monomer, but full functionality in vivo requires formation of a heteromeric complex with a ubiquitously expressed 55 kDa resident ER chaperone, protein disulfide isomerase (PDI). The 97 kDa subunit of MTTP is responsible for the lipid binding and transfer activity of the MTTP complex, while the PDI subunit contains a signature KDEL ER-retention sequence that functions to retain MTTP at the site of apoB translocation. Recent studies have revealed a role for PDI in regulating the activity of MTTP (under conditions of ER stress) and in turn modulating the ability of the liver to secrete VLDL. These findings raise the possibility that a parallel pathway may be relevant to regulating intestinal chylomicron production. MTTP is a member of the large lipid transfer protein family, including insect, nematode, and vertebrate MTTPs, the vitellogenins and also apoB. The ancestral orthologs of MTTP exhibit similar secondary and tertiary structure and interact with PDI to promote apoB secretion. Mammalian MTTP exhibits functional transfer of all lipid classes, including triglycerides, cholesteryl esters, free cholesterol, and phospholipids (PLs), but triglyceride transfer activity is highest in mammalian MTTP, perhaps providing a functional advantage in the assimilation of lipid-rich nutrients particularly during suckling. MTTP is necessary and (in the presence of apoB) sufficient for lipid export and lipoprotein secretion, as evidenced by the ability to convert nonlipoprotein secreting cell lines to apoB-lipoprotein-secreting cells when the large MTTP subunit is coexpressed with lipid-binding competent forms of apoB. On the other hand, inactivation of MTTP function using pharmacologic inhibitors or conditional genetic deletion, selectively blocks apoB-lipoprotein secretion from hepatocytes and enterocytes. Furthermore, truncating or missense MTTP mutations result in abetalipoproteinemia (ABL), an autosomal recessive disorder characterized by the absence of apoB-containing lipoproteins in serum and lipid accumulation in the liver and intestine. ABL is discussed in detail in a later section. Together, these studies have established unequivocally the role of MTTP in the assembly and secretion of triglyceride-rich lipoproteins as required steps in intestinal lipid transport.







Several studies have examined the regulation of MTTP in enterocytes, and important conclusions have emerged regarding the complexity and physiological implications for the regulation of lipid absorption and plasma lipoprotein homeostasis. Among the most intriguing findings were that intestinal MTTP exhibits diurnal regulation in concert with the diurnal changes in plasma triglyceride levels. Other findings have extended these observations with the demonstration that this diurnal regulation is mediated by CLOCK genes and requires the transcriptional repressor SHP (short heterodimeric partner). More recent studies have demonstrated that the circadian rhythm of MTTP expression may be regulated by Forkhead (FoxO) transcription factors A2 and O1 acting in concert with intestinal apolipoprotein A-IV. These findings have important implications for our understanding of how nutritional and visual cues might prime the intestine to accommodate rapid fluxes in dietary lipid availability. Leptin signaling has also been implicated in the regulation of intestinal MTTP, specifically the role of leptin receptor and melanocortin 4 receptor pathways. Yet other work has related regulation of ER stress to nutrient modulation of MTTP expression with the finding that inositol requiring enzyme 1β-knockout mice exhibit increased intestinal MTTP and augmented lipid mobilization, along with increased chylomicron secretion. More recent work has demonstrated that microRNA-30c (miR-30c) is a potent repressor of MTTP expression in mouse liver, principally by binding to regions within the 3′ untranslated region of MTTP mRNA. These authors also demonstrated miR-30C also suppressed lipogenesis and reduced diet-induced hyperlipidemia and atherosclerosis. Although miR-30C is also expressed in the small intestine as well as other tissues (including heart and testis), its role in regulating intestinal MTTP activity or lipid export from this or other tissues is yet to be determined experimentally.
49.1.4
General Model of apoB-Containing Lipoprotein Assembly
Assembly of VLDL and chylomicrons requires coordination of apoB synthesis and fusion with LDs and newly synthesized lipids in a fixed temporal sequence ( Fig. 49.2 ). A two-step model most plausibly defines the process for apoB containing lipoprotein synthesis and secretion ( Fig. 49.2 ), in which apoB is first assembled into a lipid-poor primordial lipoprotein particle in the rough ER and then acquires additional triglycerides through bulk addition of neutral lipids smooth ER to form mature triglyceride-rich lipoprotein particles. Depending on lipid availability and MTTP activity, apoB-containing lipoprotein particles may be secreted as dense VLDL, large triglyceride-rich VLDL, or chylomicrons.
49.1.4.1
First Step of Lipoprotein Biogenesis
The first step of apoB-containing lipoprotein assembly is important in governing the number of lipoprotein particles produced and is influenced by physiological variations in the rates and efficiency of secretion of newly synthesized apoB. The apoB gene is constitutively expressed, and apoB synthesis rates are relatively stable both in vitro and in vivo. For example, oleic acid supplementation in human hepatoma cells (HepG2 cells) does not affect either apoB mRNA abundance or the synthesis of apoB100, yet rapidly stimulates apoB100 secretion. Intestinal apoB synthesis is also unchanged in rat enterocytes following either acute or chronic dietary lipid challenge. Thus, the rate of secretion of apoB-containing lipoproteins is likely regulated at a posttranslational level in which (rather than altering de-novo synthesis) apoB secretion efficiency is determined by the extent to which the nascent apoB protein escapes presecretory degradation.
49.1.4.2
Presecretory Degradation of apoB
As noted above, the majority of newly synthesized apoB100 in hepatoma HepG2 cells is degraded (> 70%) cotranslationally, particularly when lipid availability is low, for example, without oleic acid supplementation, or when MTTP is either limiting or defective. ApoB degradation involves both endoplasmic reticulum associated degradation (ERAD) and the proteasomal pathway. The proteasomal degradation pathway involves ubiquitination of the nascent apoB polypeptide when it is not properly translocated across the ER membrane or becomes misfolded in the ER due to insufficient lipidation. The ubiquitinated apoB100 is transported via retrograde transport back through the ER membrane (dislocation) and degraded rapidly by proteasomal pathways. Both isoforms of apoB (both apoB 100 and apoB48) are subjected to presecretory degradation in cultured rat or mouse hepatocytes. In the liver, presecretory degradation of apoB occurs mainly through a post-ER protein degradation mechanism(s) including the LDL-receptor pathway. In contrast to the findings in hepatoma cell lines, studies in (intestine-like) Caco-2 cells suggest that intracellular apoB degradation is not detectable when MTTP activity was blocked using specific inhibitors. However, studies revealed significant amounts of apoB100 are degraded (range 70%–90%) in enterocytes isolated from Apobec-1 −/− mice, particularly with conditional intestinal Mttp deletion. ApoB48 in the enterocytes of wild-type mice was also subjected to presecretory degradation, but to a lesser extent (~ 30%) than noted for apoB100. Similarly, apoB48 is more efficiently secreted and less susceptible to presecretory degradation (even with limiting abundance or activity of MTTP) in murine hepatocytes, regardless of lipid availability. Thus, presecretory degradation may be a less important component of intestinal apoB48 secretion than was demonstrated for apoB100 secretion from hepatocytes.
49.1.4.3
Formation of Primordial Lipoprotein Particles
The assembly of apoB-containing lipoprotein particles begins with translocation of the nascent apoB peptide across the ER membrane while the polypeptide is still attached to the polysome and before protein translation of the full-length protein is complete. During this process, N-terminal domains within apoB (the first 700 residues) interact directly with MTTP to facilitate folding of the apoB polypeptide and the acquisition of neutral lipid. A number of resident ER chaperone proteins have also been demonstrated to play an important role in the folding of apoB. Formation of primordial lipoprotein particles involves release of the nascent apoB polypeptide along with lipids derived from the ER membrane, acquired through fusion with MTTP, as a protein-lipid complex into the ER lumen ( Fig. 49.2 ). This primordial lipoprotein particle contains apoB, with a PL monolayer together with limited amounts of neutral lipids. It has been suggested that apoB48 undergoes translocation into the ER lumen more efficiently than apoB100 and requires less lipidation (via MTTP) in order to become secretion competent. These differences likely reflect differences in the overall length or hydrophobicity of these two physiologic isoforms of apoB.
Independent of apoB synthesis, triglyceride-rich LDs are formed within the membrane bilayer and inside the lumen of smooth ER and this process of luminal LD assembly may represent the first step of VLDL and chylomicron biogenesis ( Fig. 49.2 ). MTTP plays a critical role in extracting both membrane-associated and other accessible pools of triglyceride and neutral lipid into the ER lumen. Maintaining these membrane pools of neutral lipids, especially triglyceride, is critical to continued intestinal lipoprotein assembly and requires replenishment by the activities of diacylglycerol acyltransferases 1 and 2 (DGAT1, 2), which are located within the ER membrane. Recent findings in mice suggest that although Dgat1 is thought to represent the dominant pathway of neutral lipid synthesis for LDs, both Dgat1 and Dgat2 each serve distinct functions in intestinal lipid metabolism and that the activity of both enzymes is required for normal lipid absorption. Earlier observations demonstrated that tubules and vesicles of a typical hepatocyte smooth ER contained LDs, suggesting that the triglyceride-rich LDs originate from the smooth ER and receive apoB synthesized from the rough ER. These findings were extended in studies of genetically engineered intestinal apoB-deficient mice. Electron microscopy examination of the intestine of these mice showed that even without apoB synthesis, there were large lipid particles present in the ER lumen. These LDs were on occasion even larger than chylomicron-sized particles, from enterocytes of wild-type mice. In other words, the ability of the enterocyte to elaborate apoB does not affect the formation of large LDs per se in the ER lumen, but rather plays a critical role in their mobilization and secretion.
By contrast, several lines of evidence support the requirement of MTTP in the formation of luminal ER LDs. For example, hepatocytes from liver-specific MTTP-deficient ( Mttp-LKO ) mice demonstrated large cytosolic triglyceride LDs, although no LDs were found within ER or Golgi compartments. Similar findings were elicited in enterocytes from intestine-specific MTTP-deficient mice ( Mttp-IKO ), where enormous LDs were present in the apical cytoplasmic compartment but no lipid particles were present within profiles of the ER or Golgi These studies strongly suggested a crucial role for MTTP in LD formation and partitioning between cytosol and ER lumen. Other findings have emphasized the dynamic formation of LDs in vivo following lipid administration along with demonstrating the presence of perilipin proteins in association with these droplets ( Fig. 49.2 ). Recent findings have demonstrated that genetic ablation of lysophophatidylcholine acyltransferase 3 (LPCAT3) in mice produces defects in intestinal LD mobilization with accumulation of intracellular lipid, morphologic abnormalities and perinatal lethality. These defects were associated with alterations in membrane PL fatty acid composition, specifically decreased arachidonyl species. These recent findings together suggest that alterations in membrane lipid FA composition may in turn influence LD formation and mobilization.
49.1.4.4
Second Step of Lipoprotein Biogenesis
After assembly of a primordial lipoprotein particle, acquisition of additional core lipid proceeds by fusion with luminal LDs. There remains some debate about how and where this so-called second step (i.e., the fusion of primordial particles and LDs) occurs. Based on earlier ultrastructural analysis of rat liver, loading of bulk neutral lipid onto nascent lipoprotein particles occurs at the junction of the rough and smooth ER, an observation which was confirmed in rabbit enterocytes. There is an evidence that newly synthesized triglycerides are preferentially incorporated into the chylomicron core, and under pathophysiologic conditions, the rates of secretion of triglyceride-rich lipoproteins may be regulated at the step of converting the smaller, denser nascent particles into larger, more buoyant particles. Among the LD-associated proteins with a possible role in coordinating intestinal LD fusion and lipoprotein assembly is the cell death-inducing DFFA-like effector B (CideB), which is associated with both cytosolic and ER-localized LDs in hepatocytes and which binds to apoB in primordial VLDL particles. Recent work demonstrated high levels of Cideb expression in small intestine, and studies showed impaired chylomicron secretion in Cideb -knockout mice, without changes in intestinal apoB secretion, suggesting a defect in the second step of chylomicron assembly. The peroxisome proliferator-activated receptor-γ coactivator-1α (PGC-1α) has been identified as a potent stimulator of TG secretion and VLDL assembly in HepG2 cells and mouse hepatocytes. Activation of PGC-1α strongly induces CideB expression in liver cells, providing a plausible explanation for its stimulatory effect on VLDL assembly and secretion. Although high-fat diet feeding increased intestinal Cideb expression, the role if any for PGC-1α in regulating intestinal Cideb expression and chylomicron secretion is unknown. Yet, other work has identified sortilin (the product of the SORT1 gene) as a novel regulator of hepatic VLDL secretion in humans that functions as an intracellular sorting receptor for apoB100 and, by interacting with apoB100 in the Golgi, facilitates export of mature VLDL particles. The potential role for these new factors in the regulation of intestinal chylomicron assembly and secretion remains to be explored.
A recent genome-wide screen for gene variants contributing to nonalcoholic fatty liver disease led to the simultaneous, independent, identification by three groups of a dominant variant in an ER-associated protein, transmembrane 6 superfamily 2, (TM6SF2). The rare TM6SF2 allele (p.Glu167Lys) was identified in association with elevated serum transaminase levels in patients with nonalcoholic fatty liver disease and was presumed to be a loss-of-function mutation that interfered with VLDL export. More recent studies using genetically modified mice demonstrated that TM6SF2 likely plays a critical role in the second step of VLDL assembly. Tm6sf2 -knockout mice revealed increased hepatic steatosis with reduced rates of hepatic VLDL-triglyceride secretion but no change in the rate of apoB secretion. TM6SF2 is mainly localized to the ER and Golgi in the hepatocytes, and its deficiency did not appear to affect intracellular LD formation. It is not understood how TM6SF2 promotes the addition of neutral lipids to the primordial VLDL particles, but it was postulated to play a role in the mobilization of neutral lipids for VLDL biogenesis. However, it is worth noting that an independent report of another Tm6sf2 -knockout mouse demonstrated no effect on hepatic triglyceride accumulation with alterations in cholesterol metabolism, suggesting that the phenotypes of these knockout lines exhibit subtle yet important differences. TM6SF2 is highly expressed in all three regions of the small intestine, with mRNA levels 10 times higher than that of the liver, although there was no apparent defect in intestinal fat absorption in those Tm6sf2 -KO mice. However, recent studies in a group of obese humans with the rare EK and KK allele indicated defective intestinal lipid secretion in response to a high fat challenge, and disruption of Tm6sf2 in zebrafish was found to perturb intestinal lipid metabolism. These apparently divergent findings with respect to the role of TM6SF2 in intestinal lipid metabolism will require further study in order to resolve a functional impact in chylomicron assembly and secretion in mammalian intestine. Along these lines, yet another line of germline Tm6sf2 -knockout mice exhibited no obvious phenotype in terms of intestinal lipid transport.
49.1.4.5
Transport of Prechylomicrons Through the Secretory Pathway
Nascent intracellular lipoprotein particles leave the ER rapidly and are transported through the secretory pathway and further modified prior to secretion. The COPII machinery has been shown to have a critical role in vesicular budding and transport of ER cargo destined for secretion through the Golgi apparatus. Studies in rat enterocytes have shown that sequential interaction of distinct pairs of heterodimeric COPII proteins (Sec 23/24 and Sec 13/31) are required for transporting the nascent prechylomicrons from ER to the Golgi apparatus. Interestingly, the liver fatty acid-binding protein (L-Fabp) has recently been demonstrated to involve in the budding of prechylomicron vesicles (PCTV) from the ER, with decreased budding and fusion in enterocyte extracts from L-Fabp −/− mice. PCTV budding was also impaired in extracts prepared from Cd36 −/− mice, suggesting a role for this multifunctional ligand receptor also. These findings are of interest since the kinetics of intestinal triglyceride secretion were significantly slower in enterocytes from L-Fabp −/− mice and kinetic delays in intestinal fatty acid transport have been found in Cd36 −/− mice. Studies in intestinal extracts suggest that COPII proteins are required for Golgi fusion but not ER budding of the prechylomicron transport vesicle. A recent study also suggest that prechylomicron and pre-VLDL are too big to fit into the conventional COPII-coated vesicle, but rather that these particles require the function of two resident ER proteins, TANGO1 and TANGO1-like (TALI) for their export from the ER. These studies demonstrated that knockdown of either TANGO1 or TALI in the intestine-like cell line Caco2 resulted in impaired secretion of apoB, which was then subject to autophagic degradation. The findings suggest that TANGO1 and TALI in human small intestine may play a key role in the terminal pathways regulating chylomicron secretion. Other studies in rat hepatoma cells demonstrated that a functional COPII complex was required for ER budding of the nascent apoB100-VLDL particle. Studies have attempted to discern the individual roles of major proteins involved in the COPII complex. Germline deletion of Sec23b resulted in neonatal lethality with systemic defects in pancreas and other secretory digestive organs. Obviously, more studies are needed to elucidate the mechanisms underlying the transport of large lipoprotein particles through the secretory pathway. Nonetheless, as discussed below, Sar1b, a member of the Sar1/Arf family of small GTPases, is an integral component of the COPII machinery. Mutations in the SARA2 gene, encoding Sar1b, have been shown to be associated with Anderson/chylomicron- retention disease (CRD), in which enterocytes are capable of assembling prechylomicrons in the ER but fail to transport them through the secretory pathway, resulting in LD accumulation in the membrane-bound compartments of the enterocytes. The mechanisms of LD formation in enterocytes are thus distinct from that in hepatocytes since CRD subjects have no defect in hepatic VLDL secretion. Recent information suggests that COPII proteins function to coordinate aspects of both lipid and cholesterol secretion from rat hepatoma cells. In addition, Sar1b deficiency decreases SREBP2 processing, suggesting that there exists a complex metabolic network of regulatory pathways linking chylomicron secretion efficiency to cholesterol homeostasis. In addition to lessons learned from CRD patients bearing Sar1b mutations, recent studies of animal and cell models have provided further evidence supporting a role of Sar1b in chylomicron secretion. Inactivation of Sar1b using antisense oligonucleotide administration in zebrafish resulted not only in increased LD accumulations in the ER and cytoplasm of enterocytes in response to a high-fat diet but also resulted in multiorgan defects associated with defective growth of the liver and pancreas. Conversely, cholesterol and oleic acid supplementation increased the expression of Sar1b in Caco-2 cells and augmented chylomicron production. In addition, transgenic overexpression of Sar1b in mice led to enhanced intestinal chylomicron secretion and increased susceptibility to diet-induced obesity and insulin resistance. Phosphorylation of Sar1b is critical in promoting prechylomicron budding from the ER, and recent studies have demonstrated that the activity of the specific zeta isoform of protein kinase C (i.e., PKC zeta) is inducible by dietary and biliary lipid, again suggesting an integrated pathway for coordinating key elements of lipid homeostasis and chylomicron assembly, transport and secretion. These concepts are summarized more extensively in a recent review.
The endocrine control of intestinal chylomicron secretion remains poorly understood, although this is an important area in view of the rising incidence of obesity and type 2 diabetes. Indeed it has been shown in normal human volunteers that short-term infusions of glucose result in increased intestinal lipoprotein secretion, suggesting the hyperglycemia and poorly controlled diabetes may result in elevated intestinal lipoprotein secretion and contribute to the metabolic consequences of diabetes. Earlier work showed that glucagon-like peptide 2 (GLP-2) stimulated intestinal chylomicron export by binding to receptors expressed on L cells which in turn generated mediators including insulin-like growth factor 1 (IGF1) which signals via receptors expressed on enterocytes. The signaling mechanisms also include altered fatty acid transport mediated via the multiligand transporter CD36 since the enhancement of intestinal chylomicron secretion produced by GLP2 was attenuated in Cd36-knockout mice. More recently, studies have demonstrated that GLP2 signaling involves a nitric oxide (NO)-dependent mechanism since pharmacologic inhibition of NO mitigated the effects of GLP2 as did studies conducted in genetically altered mice unable to synthesize NO. Among the key observations was that NO treatment resulted in increased Mttp activity and promoted secretion of triglyceride from preformed intracellular stores.
49.2
Genetic Defects in APOB and MTTP
49.2.1
Familial Hypobetalipoproteinemia
Familial hypobetalipoproteinemia (FHBL) is an autosomal codominant disorder characterized by low (below the 5th percentiles for age and sex) plasma concentrations of total cholesterol, LDL cholesterol, and apoB. FHBL is clinically heterogeneous, but most cases are caused by nonsense and frameshift mutations in the APOB gene that lead to formation of C-terminal-truncated apoB. Truncated apoB variants are designated according to a centile nomenclature with numerous different mutations in the apoB gene, producing mutant APOB alleles ranging from apoB0.5 to apoB89. The frequency of APOB gene mutations causing truncated apoB proteins is approximately 1:3000, but it has been estimated by clinical criteria that the frequency of heterozygous FHBL is 1:500–1:1000. On the other hand, the incidence of homozygous FHBL is very rare. A number of missense APOB mutations have been reported as a cause of FHBL, all of which are distributed within the amino-terminal 1000 amino acid residues. Unlike the truncated proteins arising from APOB variants, which are secreted efficiently, the products of amino-terminal APOB missense mutations are secreted poorly and are retained in the ER and/or Golgi compartments and degraded by proteasomal and autophagosomal mechanisms. Heterozygous FHBL subjects are often asymptomatic but have plasma LDL-cholesterol and apoB concentrations that are one-fourth to one-third of normal. Low plasma cholesterol levels appear to be well tolerated and not associated with defects in intestinal fat absorption. The clinical and biochemical features of homozygous FHBL, by contrast, are similar to that noted for subjects with homozygous ABL. Homozygous FHBL patients may have deficiencies in fat-soluble vitamins secondary to fat malabsorption as a result of defective chylomicron production. The clinical features of both ABL and FHBL have been extensively reviewed recently in Ref. .
49.2.1.1
Mechanisms Underlying the Phenotype With FHBL
Truncated apoBs smaller than apoB27.6 lack intrinsic lipid-binding properties and are usually undetectable in plasma. Evidence from in vitro studies demonstrates that most of these shorter mutants are secreted efficiently from rat hepatoma cells. However, these truncated apoB variants (particularly smaller than B70) are not stable in the plasma and are rapidly cleared. Thus, even when detectable in FHBL subjects, truncated apoBs are found at very low concentrations, usually 10%–30% of apoB100 encoded from the normal allele in FHBL heterozygotes.
Another unexpected feature of FHBL is that plasma levels of apoB100 in heterozygous FHBL individuals are much lower than the 50% predicted based on the preservation of one normal apoB100 allele. Lipoprotein kinetic studies of the heterozygotes FHBL humans indicate that production rates of apoB100 are reduced by 70%–80%, instead of the expected 50%. Thus, the presence of a premature-termination mutation in one apoB allele somehow impairs the synthesis and/or secretion of apoB100, the gene product of the unaffected allele from the liver. A corollary of the low production rates of apoB100-VLDL in the setting of FHBL is the association with hepatic steatosis, and studies have shown that heterozygous FHBL subjects with a range of apoB truncations (apoB4 to apoB89) have hepatic triglyceride content threefold higher than control individuals. Presumably, the reduced hepatic triglyceride-exporting capacity plays a major role in the development of fatty livers in these subjects. It is important to emphasize that because of apoB mRNA editing activity in the intestine, apoB truncations larger than apoB48 would not be likely produced by the small intestine. Accordingly, the liver is responsible for the production of truncated apoBs larger than apoB48, whereas both liver and intestine contribute to the lipoproteins particles bearing truncated apoB variants smaller than apoB48.
Most studies of FHBL subjects have focused on derangements of plasma and hepatic lipid metabolism and relatively little is known about the effect of apoB-truncation mutants on intestinal lipid metabolism. The currently available data indicate that intestinal fat absorption and secretion are minimally affected in FHBL heterozygotes. One such study examined 10 heterozygous FHBL patients with apoB truncations larger than apoB48 (apoB-89, -75, -54, and -52), 6 heterozygous FHBL patients with apoB truncations smaller than apoB48 (apoB-46, -40, and -31), and 16 controls found no difference in response to a constant vitamin A oral fat loading test between the FHBL heterozygotes and the control subjects, indicating that one allele specifying the intestinal production of apoB48 may be sufficient for normal fat absorption. However, other studies have demonstrated intestinal fat accumulation and reduced postprandial lipemia in heterozygous FHBL subjects with APOB R463W missense mutation, suggesting that this missense mutation impairs the ability of apoB48 (produced from the normal APOB allele) to assemble and secrete chylomicrons.
49.2.1.2
Mouse Models of FHBL
Researchers have generated several lines of apoB gene-targeted mice, including Apob -null mice and mice bearing a range of truncations. These lines of mice display a range of phenotypes reflective of FHBL, including low plasma apoB100 levels with truncated apoB-containing lipoproteins and hepatic steatosis. Studies of these mice have revealed an essential role for apoB in nutrient transport during embryonic development in rodents, with embryonic lethality during mid-gestation due to the inability of the visceral yolk sac endoderm to produce sufficient triglyceride- rich lipoproteins to support critical functions, particularly nervous system and neural tube development. These developmental defects in mice are not observed in humans with FHBL.
The apoB38.9-bearing mouse was particularly valuable in understanding features of the FHBL syndrome because it faithfully replicated the same single nucleotide mutation of the APOB found in humans without introducing extra alterations to the mouse apoB gene. Even so, this mutation still reduced the steady-state mRNA levels of the affected apoB allele by 40% in the liver and intestine, providing direct evidence that reduced mRNA expression of the mutant apoB gene could be an important mechanism for the low production rates of truncated apoB variants in FHBL humans. ApoB38.9 was secreted efficiently by hepatocytes, but mainly as triglyceride-poor, HDL particles, whereas apoB48 was secreted mainly as VLDL and IDL particles. These results demonstrate that deleting the C-terminal 400 amino acid residues for apoB48 greatly reduces its ability to assemble into triglyceride-rich lipoproteins. Due to the inability of apoB38.9 to assemble triglyceride-rich lipoproteins, the apoB38.9 mice have defective hepatic triglyceride secretion and develop fatty liver. To investigate the mechanism underlying the larger-than-expected decrease in apoB100-VLDL production in the FHBL heterozygotes, the apoB38.9 mice were crossed with apoB100-only mice or Apobec-1 −/− mice in order to eliminate apoB48 formation in the liver and small intestine. Findings from this cross demonstrated reduced hepatic apoB100 secretion rates in mice carrying the apoB38.9 mutation, specifically a 70%–80% reduction in apoB100 secretion, as compared to control mice in which both Apob alleles specify apoB100 production. These data suggest that the Apob mutation in FHBL heterozygotes may affect the secretion of apoB100 from the wild-type Apob allele in a posttranslational manner. Like heterozygous FHBL subjects, the apoB38.9-heterzygous mice exhibited no defects in intestinal fat absorption while the mice consume a chow diet. However, when these mice were fed a high-fat diet, they displayed reduced fat absorption capacity. Despite the reduced fat absorption, these mice were still more susceptible to diet-induced hepatic steatosis than wild-type littermate controls, resulting from the impairment in hepatic TG export as discussed above.
49.2.2
Abetalipoproteinemia
Mutations in the structural gene encoding MTTP on chromosome 4q22–24 cause ABL, a rare autosomal recessive disorder characterized by virtual absence of apoB-containing lipoproteins in blood. ABL typically presents as failure to thrive in infancy due to fat malabsorption, or in older children as an atypical pigmented retinopathy associated with spinocerebellar degeneration. The clinical manifestations of homozygous ABL were described by Bassen and Kornzweig more than six decades ago and have been recently summarized. In fasting patients, plasma cholesterol levels are very low (~ 50 mg/dL), and triglyceride levels are typically < 10 mg/dL. Even after a fat load, chylomicrons and apoB48 do not appear in plasma. In conjunction with the plasma findings, ABL subjects manifest massive neutral lipid accumulation in both enterocytes and hepatocytes. Most obligate heterozygotes (~ 80%) have normal plasma lipid levels, but a small subset of ABL heterozygotes may have relatively low cholesterol levels; the mechanisms underlying this phenotypic heterogeneity is not fully understood.
The clinical manifestations of ABL reflect a block in the secretion of apoB-containing lipoproteins. An established link between MTTP expression and ABL was confirmed by demonstrating that both the large 97-kD subunit of MTTP and triglyceride transfer activity were absent in intestinal biopsies from subjects with homozygous ABL. Most of the MTTP mutations result in the formation of premature-termination codons and the production of C-terminally truncated forms of MTTP, indicating a critical functional role for the C-terminal region in apoB-lipoprotein assembly. Recent studies have identified additional missense mutations in the MTTP gene associated with low levels of plasma triglyceride, including a mutant D361Y allele in which the expressed protein was unable to bind to the chaperone PDI and consequently was unable to function in either lipid transfer or to support apoB secretion.
49.2.2.1
Mouse Models of ABL
Understanding the tissue-specific functions of MTTP in a murine model has obvious appeal as a tool to probe the regulation and physiological functions of this gene. The initial approach of using germline Mttp deletion, however, produced embryonic lethality with fetal demise around embryonic day 10. As noted above, the same phenotype of embryonic lethality was observed in mice with germline Apob deletion, suggesting that both MTTP and apoB are required for normal murine fetal development. In contrast, heterozygous Mttp +/− mice showed a somewhat surprising phenotype in that MTTP activity and mRNA were reduced by 50% in both the liver and intestine, and hepatic apoB secretion was also reduced by 20%–30%. Thus, mice with one targeted Mttp allele appear to manifest an autosomal dominant trait, in that elimination of one allele reduced the function of the gene by ~ 50%. Humans with heterozygous MTTP deletions by contrast have normal MTTP activity in the intestine and manifest an autosomal recessive trait. As noted above, although decreased plasma cholesterol is occasionally found in heterozygous ABL subjects, the majority of such subjects do not manifest hypocholesterolemia (reviewed in Ref. ).
Advances in understanding the tissue-specific role of MTTP in lipoprotein assembly have been facilitated by the development of conditional tissue-specific-knockout mice. Inactivation of hepatic Mttp ( Mttp-LKO ) dramatically reduced lipoprotein secretion although an important discrepancy was noted between these studies. One group reported near absent hepatic secretion of both apoB100 and apoB48 in Mttp-LKO mice, while another group reported virtual elimination of apoB100 secretion with unaltered apoB48 secretion. These two groups used different gene targeting strategies, although both effectively eliminated lipid transfer function, and the discrepancy in differential sensitivity for apoB100 versus apoB48 secretion remains unresolved. However, it is relevant that, when applied to cultures of primary murine hepatocytes, pharmacologic MTTP inhibitors produce much greater inhibition of apoB100 than of apoB48 secretion, implying that there exists a differential sensitivity to the requirement for lipidation of apoB100 versus apoB48.
Since ABL is characterized by gross lipid accumulation in both hepatocytes and enterocytes of the small intestine, the findings from intestine-specific Mttp deletion ( Mttp-IKO ) have special relevance, particularly in view of the findings above suggesting that apoB48 secretion was relatively unimpaired in at least one Mttp-LKO model. Mttp-IKO mice manifested dramatic lipid engorgement of small intestinal enterocytes with massive cytoplasmic LD accumulation, findings that phenocopy the observations in humans with ABL. In addition, secretion of apoB48 from isolated enterocytes was decreased in Mttp-IKO mice, suggesting that apoB48 secretion is indeed regulated by Mttp function. Further studies have illuminated the tissue-specific role of Mttp in relation to ApoB isoform expression in mouse small intestine. As reviewed above, a posttranscriptional modification mediated by an RNA-specific deaminase, Apobec-1, leads to the production of apoB48 in small intestine. When the Mttp-IKO mice were crossed into an Apobec-1 −/− background (to yield apoB100-only Mttp-IKO mice), these animals demonstrated dramatic lethality as a result of intestinal lipotoxicity, particularly when fed a high-fat diet. The findings further demonstrated an induction of the unfolded protein response and ER stress in the compound ( Apobec-1 −/− , Mttp-IKO ) background, suggesting an important and heretofore unappreciated element of the tissue-specific interactions of Mttp and apoB. The findings in the compound line alluded to above have particular implications for understanding the role of MTTP deletion or pharmacologic inhibition in humans, which are known to produce hepatic biochemical abnormalities and elevated transaminase levels. These findings contrast with results from pharmacologic inhibitors in mice, where no dramatic alterations in ER stress were found in the liver. However, more recent studies have demonstrated that liver-specific Mttp -knockout mice actually develop hepatic fibrosis, suggesting that the accumulation of hepatic lipid is not benign. Those findings in mice replicate observations in humans with ABL where advanced fibrosis has been observed.
Shared features of the conditional tissue-specific Mttp -knockout lines offer insights into the role of MTTP in the first versus second step of lipoprotein biogenesis. Mttp-LKO mice demonstrated disappearance of VLDL-size lipid particles within the ER and Golgi providing in vivo evidence for a role of MTTP in promoting formation of apoB-free lipid particles in the ER. A similar phenotype was observed in Mttp-IKO mice (i.e., absence of lipid particles within profiles of the ER and Golgi), suggesting that while apoB48 secretion was not completely blocked, the second step of VLDL assembly was inhibited, producing severe impairment in triglyceride export and intracellular lipid accumulation.
Recent work has extended the impact of disrupting small intestinal Mttp function by demonstrating that intestine-specific Mttp -knockout mice exhibit a survival advantage in the setting of systemic sepsis with reduced mortality and preservation of intestinal proliferation. These findings raise the possibility that strategies to impede intestinal chylomicron secretion might improve the outcomes associated with systemic sepsis. Further work demonstrated that this protective effect was lost in aged, intestine-specific Mttp -knockout mice, despite lower serum levels of cytokines including TNF alpha and without obvious differences in the levels of bacteremia. Taken together, the findings suggest that there is a temporal component to the protective effects of blocking chylomicron export on the response to systemic sepsis. Other findings have demonstrated that intestine-specific Mttp-knockout mice exhibit more colonic injury following dextran sodium sulfate ingestion and demonstrate increased tumor burden in the setting of colitis-associated cancer. These findings raise the question of how dietary fat intake and altered intestinal lipid handling might influence pathways relevant to the pathogenesis of colorectal cancer.
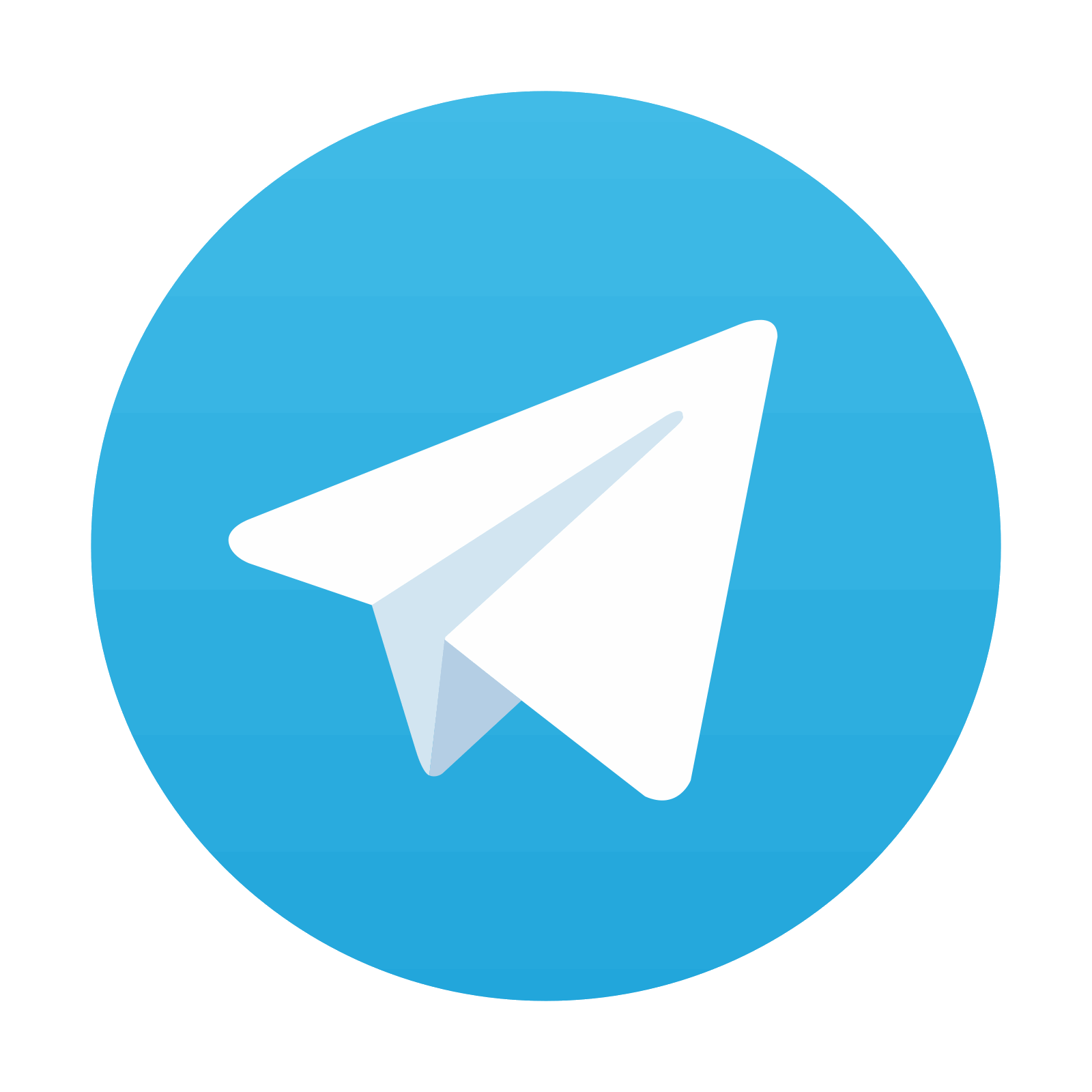
Stay updated, free articles. Join our Telegram channel
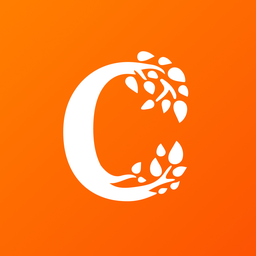
Full access? Get Clinical Tree
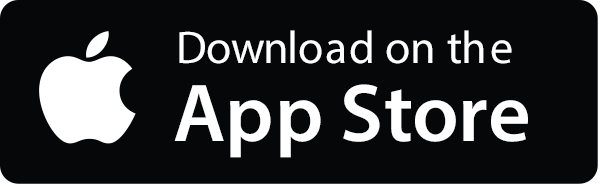
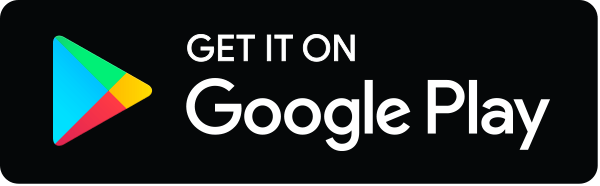