Introduction
In recent years, the number of gene therapy (GT) trials targeting the liver have grown significantly, particularly when it comes to gene addition strategies (delivery of cDNA for a whole gene to the target organ/cells, which then exists in addition to the host genome with the mutated gene) to rescue monogenic diseases. Although several gene delivery approaches have been tested in preclinical animal models, including viral and nonviral approaches, adeno-associated virus (AAV) vector-based gene transfer remains the most popular gene delivery platform for the liver, with a few trials entering phase III. Excellent safety profile, high efficiency of transduction of hepatocytes, and evidence of long-term efficacy deriving from early-phase trials have established AAV vectors as the platform of choice for the treatment of enzyme deficiencies and metabolic disorders. The large number of proof-of-concept studies in animal models showing therapeutic efficacy following AAV vector gene transfer is evidence of a potentially rich pipeline of GT drugs that could potentially be brought to the clinic and change the way genetic diseases could be treated, particularly liver diseases.
Clinical translation of novel therapies, however, is a process that involves several bench-to-bedside cycle iterations, during which issues associated with the technology are encountered and solved. For the AAV vector gene transfer technology, several hurdles have been highlighted both in preclinical studies and clinical trials; addressing these issues will contribute to expanding the number of indications in which clinical success can be achieved.
In this chapter, we will discuss some of the key approaches to GT for liver-based metabolic diseases (LBMDs), with a focus on the advances of gene addition approaches and AAV-based GT strategies, and will present some of the main achievements, and emerging issues, of the field of in vivo gene transfer. These emerging issues are:
- 1.
AAV vector and transgene immunogenicity;
- 2.
genotoxicity risk associated with AAV gene transfer to the liver;
- 3.
AAV gene transfer persistence in a developing versus adult liver.
Why Gene Therapy? What Is the Advantage Compared With Liver Transplantation? Pros and Cons for Liver Transplantation and Gene Therapy
Inherited diseases are a substantial burden of childhood disease, and they result in up to 70% of all admissions to children’s hospitals. Because most metabolic activity in the body happens in the liver, and furthermore, most metabolic diseases affect, at least partly, the liver, a significant subgroup of inherited diseases is the group of LBMDs. In some conditions, all of the defective or deficient enzymes are expressed in the liver; in others, they are partially expressed in the liver and partially in other organs such as muscle, kidney, or central nervous system ( Table 33.1 ). In many LBMDs, the livers themselves are not diseased ( Table 33.1 ).
Expression Mainly From Hepatocytes/Only Hepatocyte-Based Pathway | Expression Also in Other Organs/Impaired Pathways in Other Organs | |
---|---|---|
With progressive liver disease/fibrosis
| Group A | Group B |
Without or slow progressive liver disease if treated
| Group C
| Group D
|
a Cumulative incidence, 1:50,000–100.000.
b Cumulative incidence, 1:20,000. BSEP , Bile salt export protein; HCC, hepatocellular carcinoma.
GT could cure or at least treat inherited diseases and is much more curative than other treatment options so far while having the potential to have fewer side effects and burden for the patient compared with liver transplantation (LT). In addition, LT treatment is often limited to supportive measures and may entail significant adverse effects, long-term organ failure, risk of metabolic crises, and malignancy or impairment in the quality of life. Especially for LBMDs without structural damage in the liver ( Table 33.1 , group C and D), the LT is a rough kind of “gene correction.” Even though LT for LBMDs has an excellent long-term outcome, with 5-year survival above 90%, the procedure is associated with significant morbidity and mortality. The benefits and risks of GT compared with LT are different depending on disease and disease group, corresponding to Table 33.1 and like those shown in Table 33.2 . In Table 33.3 , general advantages and disadvantages of GT (exemplary for nonintegrating or targeted integrating recombinant AAV [rAAV] GT approaches) versus LT are shown.
Expression Only From Hepatocytes/Only Hepatocyte-Based Pathway | Expression Also in Other Organs/Impaired Pathways in Other Organs | |
---|---|---|
With progressive liver disease/ fibrosis
| Group A
| Group B
|
Without progressive liver disease
| Group C
| Group D
|
Gene Therapy | Liver Transplantation | |
---|---|---|
Concept | Only correcting defect gene in healthy liver | Replacement of a healthy liver to correct one gene |
Experience, knowledge | Less experience, unknown long-term outcome | Established treatment, good long-term outcome |
Safety | Risk of insertional mutagenesis, off-target effects | Perioperative mortality, mortality on waiting list |
Efficiency | Not all hepatocytes will be corrected; correction of some hepatocytes is usually sufficient for phenotypic correction | All hepatocytes were replaced |
Stability/persistence | Depending on the approach and age of the patient | Usually lifelong, but need for liver retransplantation because of chronic rejection or other problems is possible |
Patient selection | So far, only feasible in patients without preexisting antibodies (new approaches to circumvent this are under progression/evaluation). Problems with stability/persistence in patients under 10 years. | Feasible at all ages, but risk of mortality, morbidity, and long-term side effects of immunosuppression |
Immunosuppression | No or short-time immunosuppression | Lifetime immunosuppression with side effects such as elevated risk for tumors and kidney dysfunction |
Transgene antibodies | Many studies have shown that rAAV-based liver-directed gene therapy causes immunological acceptance of transgene | Evident in some diseases, for example, BSEP deficiency (PFIC2) |
Application | Portal vein or peripheral vein vector infusion | Substantial surgery |
Resources | Unlimited resources | Organ shortage |
Timing | Therapy at every time, no waiting list | Cannot be influenced, depending on organ offers |
For diseases with cell-autonomous behavior like primary hyperoxaluria and/or defects not only evident in the liver ( Table 33.1 , groups B and D), for example, propionic academia, GT is more difficult, especially when the aim is to transduce the other organs also, but at the same time it has the potential to deliver a treatment that is much closer to cure than LT, where only the defect in the liver will be treated. The first promising results for this are shown in propionic academia.
The Clinical Experience With Liver Gene Transfer
The liver is a particularly attractive organ for the development of gene-based therapeutic approaches because it presents several attractive organ-specific features, which include (1) the fact that it is one of the body’s major biosynthetic organs, which confers an advantage when it comes to turning hepatocytes into biosynthetic units; (2) the liver has a unique dual blood supply from the hepatic portal vein and hepatic arteries and a fenestrated endothelium, and thus hepatocytes can be relatively easily targeted with both viral and nonviral vectors; (3) despite the predominantly nonintegrative nature of AAV vectors, owing to the relatively slow turnover of mature hepatocytes, multiyear transgene expression after gene transfer to the liver has been documented in large animals and humans; (4) expression of a transgene in hepatocytes induces antigen-specific tolerance mediated by regulatory T-cells (Tregs); and (5) several preclinical studies demonstrate that it is possible to treat not only plasma protein deficiencies but also metabolic disorders with liver gene transfer, resulting in a long-term cure for many of these disorders in small and large animal models.
Liver gene transfer with AAV vectors has been tested in the clinic in the context of few indications, although the initial evidence of success obtained with liver gene transfer for hemophilia B paved the way for a number of clinical studies of AAV liver gene transfer ( Table 33.4 ). Initial results in the dog model of hemophilia B provided a strong rationale for targeting the liver to express the therapeutic FIX transgene. In the first AAV-FIX liver trial, a single-stranded AAV2 vector carrying the human FIX transgene expressed under the control of a liver-specific promoter was administered through the hepatic artery. This trial has been particularly important for the field of in vivo gene transfer because it demonstrated for the first time that it was possible to transduce the human liver with AAV vectors, leading to therapeutic levels of transgene expression. Additionally, it allowed the identification of important limitations of the approach related to vector immunogenicity and preexisting immunity to AAV in humans. Following the results obtained in the AAV2-FIX trial, a second trial was initiated in which a self-complementary AAV8 vector encoding for a codon-optimized version of the FIX transgene was administered intravenously to target the liver of hemophilia B subjects. In this study, a short course of immunosuppression was used to block potentially detrimental immune responses triggered by the viral vector, an approach also adopted in other GT trials. This initial trial successfully demonstrated that it was possible to target the liver via the administration of an AAV8 vector delivered through a peripheral vein. Additionally, it showed that transient immunosuppression could be safely applied with gene transfer to avoid detrimental immune responses, leading to long-term expression of the transgene product.
Sponsor | Indication | AAV Serotype – Transgene | Stage (NCT Identifier) | |
---|---|---|---|---|
University College London and Saint Jude Children’s Research Hospital | Hemophilia B | AAV8 – human FIX | Phase I/II (NCT00979238) | |
Spark Therapeutics and Pfizer | Hemophilia B | AAV-Spark100 – human FIX Padua | Phase I/II (NCT02484092; NCT03307980) | |
UniQure | Hemophilia B | AAV-5 – human FIX Padua | Phase III (NCT02396342) | |
Freeline Therapeutics | Hemophilia B | AAV-FLT180a – human FIX Padua | Phase I/II (NCT03369444) | |
Biomarin | Hemophilia A | AAV-5 – human factor VIII BDD | Phase III (NCT02576795; NCT03392974; NCT03370913) | |
Spark Therapeutics | Hemophilia A | AAV-Spark200 – human factor VIII BDD | Phase I/II (NCT03003533; NCT03432520) | |
University College London and Saint Jude Children’s Research Hospital | Hemophilia A | AAV-8 – human factor VIII v3 | Phase I/II (NCT03001830) | |
Sangamo and Pfizer | Hemophilia A | AAV-5 – human factor VIII BDD | Phase I/II (NCT03061201) | |
Shire | Hemophilia A | AAV-8 – human factor VIII BDD | Phase I/II (NCT03370172) | |
Ultragenyx and Bayer | Hemophilia A | AAV-8 – human factor VIII BDD | Phase I/II (NCT03588299) | |
Audentes Therapeutics | Crigler-Najjar syndrome | AAV-8 – human UGT1A1 | Phase I/II (NCT03223194) | |
Genethon | Crigler-Najjar syndrome | AAV-8 – human UGT1A1 | Phase I/II (NCT03466463) | |
Adverum | Alpha 1 antitrypsin deficiency | AAV-Rh10 – human AAT | Phase I/II (NCT02168686) | |
Federico II University | Mucopolysaccharidosis type I | AAV-8 – human ARSB | Phase I/II (NCT03173521) | |
Ultragenyx | Ornitine transcarbamylase deficiency (OTC) | AAV-8 – human OTC | Phase I/II (NCT02991144) | |
Ultragenyx | Glycogen storage disease type 1a | AAV-8 – human G6PC | Phase I/II (NCT03517085) |
Delivery Tools (Vectors) With Advantages and Disadvantages for Each
Several gene delivery tools are available to transfer a transgene expression cassette to hepatocytes. These include both viral and nonviral vectors ( Table 33.5 ). The AAV vector platform is currently the most popular platform for liver gene transfer, although recent advances in manufacturing and encouraging results in animal models of hemophilia with lentiviral vectors have been documented. Owing to advances in the technology and potential benefits in terms of lack of preexisting immunity and theoretical ability to redose, nonviral delivery methods have recently gained attention as attractive tools for in vivo gene transfer to the liver. Both lentiviral vectors and nonviral delivery methods are currently being tested in animal models as tools for liver gene transfer for diseases like hemophilia and liver metabolic diseases, respectively.
Vector | Pros | Cons |
---|---|---|
Nonviral vectors (based on nanoparticles) | Easy to manufacture; can accommodate large DNA fragments; no preexisting immunity; repeated dosing theoretically possible. | Lower efficiency compared with viral vectors; nuclear transport of genetic payload; persistence of transgene expression. |
Lentiviral vectors | Low preexisting humoral immunity; persistence of transgene expression in dividing cells; induction of tolerance to the transgene. | Can transduce immune cells (dendritic cells, etc.); integrational mutagenesis; manufacturing can be challenging. |
Adeno-associated virus vectors | Target the liver efficiently; can be manufactured in large amounts; do not integrate efficiently into the host genome; induction of tolerance to the transgene. | Preexisting immunity to the vector capsid; transgene expression does not persist in growing liver; vector readministration is challenging. |
Advantages and Disadvantages of Different Gene Therapy Approaches (Gene Repair, Gene Addition, Gene Editing, Homologous Recombination, Nucleases, Promotorless)
For interpretation of clinical and preclinical studies as well as for discussing GT as a curative treatment for an LBMD, it is important to evaluate GT disease specifically and to differentiate between the multiple options of GT that have been described so far ( Figure 33.1 ). For this, it is also important to be aware of the stage of transition from preclinical to clinical applications for different methods. Next, we describe the methods that are already used in clinical studies or closest to this or that are the most important/innovative. Figure 33.1 gives an overview for this.

First, in vivo and ex vivo GT must be differentiated. For clinical purposes, especially for LBMDs, ex vivo GT is less close to clinical transition.
Second, which vector (e.g., AAV) or kind of application (e.g., naked DNA, nanoparticle, exosome) is used (see also Table 33.5 ) has to be differentiated. For gene addition, the vector can also determine if the transmitted cDNA is integrated or not. A very recent study described a new option for GT with naked DNA and hydrodynamic injection, which was so far not suitable for clinical translation. But Khumbari et al. described a hydrodynamic injection in the biliary system via endoscopic retrograde cholangiopancreatography, which could overcome the problems hydrodynamic injection into blood would cause in humans.
Third, the architecture of the transmitted genetic information can be different, for example, a whole gene with or without flanking homologous arms for a specific area on the host genome or just parts of a gene for gene repair; furthermore, different promotor or enhancing sequences or codon optimizations can be added.
Fourth, there is the possibility to add tools to the introduced genetic information, such as nucleases (e.g., clustered regularly interspaced short palindromic repeat [CRISPR]/CRISPR-associated protein 9 [Cas9]) to facilitate targeted integration.
Different combinations from these four aspects are possible and also depend on the kind of GT that is intended. This can be gene repair, gene silencing, gene addition, or RNA splicing manipulation approaches. Table 33.6 provides a short overview of the different approaches and their characteristics, and the corresponding Figures 33.2–33.5 describe the approaches graphically.
Approach | Description | Pros | Cons | Development stage |
---|---|---|---|---|
Gene addition -Episomal ( Fig. 33.2 ) -or random %integration -or targeted integration (HDR ± nucleases, e.g., Cas9) ( Fig. 33.3 ) – or Promoterless/ GeneRide strategy ( Fig. 33.4 ) | Classic rAAV GT, easy to apply most expertise, can be enhanced by new techniques Retrovirus GT, lentivrus GT rAAV GT with homologous sequences added to the template DNA, integration via HDR at safe harbor locus (e.g., ROSA 26), can be enhanced with DSB caused by nucleases A new approach that uses the idea of targeted integration but instead of safe harbor locus, an organ-specific gene with high expression rate is targeted, so that the template DNA must not have a promotor. This approach can be performed with or without nucleases | Low risk of insertional mutagenesis or off-target effects Very stable transgene expression Very stable transgene expression also in the growing liver of young children, very low insertional mutagenesis, low off-target/random integration events (especially without nucleases) Very organ specific, use of the natural promotor, low insertional mutagenesis | Loss of template DNA/transgene expression because of cell turnover in young children (< 10 years) Risk of insertional mutagenesis Low efficiency (for no-nuclease approach); for nuclease approach, two vectors have to be applied and risk of off-target DSB Risk of disruption or mutation of targeted gene in case of nuclease application, low efficiency in case of no nuclease application | Clinical studies are done and running (CureCN), most translational approach at the moment, possibilities for readministration are under evaluation Nathwani et al. Meliani et al. not suitable for translation at the moment Rittelmeyer et al. very attractive approach and well working in animal studies, but there are still safety concerns for translation Junge et al. Landau et al. Very new and attractive approach but still far from translation Porro et al. Sharma et al. |
Gene editing/repair HDR ± nucleases ( Fig. 33.5 ) | In case of a small or point mutation, this part can be replaced by a correct template sequence with homologous arms. Again, efficiency without nucleases is low. | Very low risk for mutagenesis, using natural promotor for the specific gene. | In case of NHEJ instead of HDR events, a reserved activity of the mutated gene can be erased so that disease phenotype is declined. Only applicable for diseases with frequent mutation. Nucleases have to be designed for each disease or even different mutations. | Only applicable for a portion of diseases. Yang et al. 166 |
Ex vivo | Hepatocytes, isolated by partial hepatectomy in the patient, are treated in vitro with gene therapy vectors; for this, all abovementioned approaches are possible. | Hepatocytes can be targeted directly, no side effects in other organs, lower vector doses are necessary. | Allogenic hepatocytes no option, autologous hepatocytes need a partial hepatectomy in a sick or small or liver-diseased patient. | Still focused on animal studies, extraction of hepatocytes is a problem VanLith et al. |




Disease-Specific Aspects and Milestones
Next, we will describe exemplary some disease-specific aspects and suitability for GT as well as important disease-specific study results. Fig. 33.6 gives an overview of disease-specific suitability for GT.

Crigler-Najjar Syndrome
Crigler-Najjar syndrome (CNS) is a recessive inherited disorder caused by a deficiency of uridine diphosphoglucuronosyl transferase 1A1 (UGT1A1). Because of missing hepatic bilirubin glucuronidation, patients experience accumulation of unconjugated bilirubin in serum, leading to a risk of irreversible damage to the central nervous system. For CNS, a natural mutant rat model exists, the Gunn rat, and also a transgenic mouse model was described. The mouse model requires phototherapy for survival to adulthood, whether or not it’s the Gunn rat. The CNS is an ideal model for GT because liver parenchyma in classical CNS is considered structurally and histologically normal; however, it is reported that over time, some fibrosis can develop. Further, the correction level needed for sufficient phenotypic correction is low—only 5% of normal UGT1A1 activity. Finally, the success of the intervention is easily monitored by serum bilirubin levels. Up to now, the only available conservative therapy to individuals affected by the severe form of CNS is phototherapy, which has major implications on the quality of life and loses efficacy during adolescence. LT during adolescence is often considered to be the only curative option available to prevent acute decompensation and irreversible brain damage. For CNS, various GT approaches have been tested in animal models. The rAAV approach seems to be the most promising. Seppen et al. showed a 70% reduction of serum bilirubin levels following AAV gene transfer for UGT1A1. The dose used in this study (2.5–5 × 10 12 vector genomes [vg]/kg body weight) was similar to that used in the high-dose cohort of the rAAV8 trial of GT for hemophilia B, which showed efficacy, although with detection of immune responses against the viral capsid in some patients. In the UGT1A1 knockout mouse model, Bortolussi et al. were able to show phenotypic correction after intraperitoneal injection of AAV9 UGT1A1 vectors in neonate mice.
More recent work in Gunn rats shows that AAV8-mediated gene transfer to the liver using an optimized UGT1A1 expression cassette results in correction of bilirubin levels at doses lower than those tested so far, and also, studies in the mouse model showed sufficient therapy with 2.5 × 10 11 vg/kg body weight.
Like in many LBMDs, it would be suitable to treat the disease in children, first, to eliminate the risk for neurological damages, second, to avoid the quality of life impairing phototherapy as early as possible, and third, to avoid development of liver fibrosis (based on very recent findings ). But this is difficult because of the described problem of transgene-loss in rAAV8 episomal GT in the growing liver.
Two recent studies tested the readministration in the Crigler-Najjar mouse model with success. Greig et al. readministered the same vector on day 56 after first injection within the first 24 hours of life. Because of the very early first injection, mice did not develop antibodies, which is also described by Wang et al. However, this approach is very unlikely to be transferred to the clinic because such an early diagnosis in the newborn is impossible except when genetic testing is performed in pregnancy because of known risk. More translational is the approach of Bockor et al., with serotype switching for readministration. For this approach, the need for the approval of two products could hamper clinical translation. Better solutions could be to find possibilities for readministration or targeted integration (see “AAV Persistence in the Developing Liver”). One very attractive targeted integration approach was studied in the Crigler-Najjar mouse model by Porro and colleagues. They could reduce bilirubin levels in the mice by intraperitoneal injection of rAAV2/8 packed with a template DNA consisting of homologous arms for the albumin locus so that UGT1A1 cDNA is integrated into the host genome at the end of the albumin reading frame separated from the albumin by a 2a peptide. This approach reached 5% enzyme activity of the wild type, enough for significant reduction of serum bilirubin levels, without any nuclease-enhancing homology-directed repair/homologous recombination (HDR) but with high vector doses (1 × 10 12 vg/mouse). Therefore for clinical translation, this approach needs to be optimized.
Glycogen Storage Disease Type 1
Glycogen storage disease type 1a (GSD1a) is caused by glucose 6-phosphatase (G6Pase) deficiency, which is expressed mainly in the liver, the intestines, and the kidney. Long-term complications of GSD1a include life-threatening hypoglycemia, failure to thrive, renal failure, osteoporosis, and hepatic adenomas and carcinomas. Patients with GSD1b additionally suffer from neutropenia and myeloid dysfunction. Dietary therapy has significant limitations, and patients are constantly exposed to the risk of life-threatening hypoglycemia. In some metabolic diseases, protein/enzyme replacement is a treatment option, but this option is expensive, needs high-frequency readmissions, and can be hampered by antibody production. For GSD1, this is no option because the defect G6Pase is an extremely hydrophobic transmembrane protein that is difficult to purify. Different animal studies for GT in GSD1a have been performed with different results. Most show a good metabolic correction and assume prevention of hepatocellular carcinoma (HCC), but others show a decline of transgene expression over time and still a risk for HCC. In the following, some of these studies are summarized.
Luo et al. showed in their study that the mouse model can reach metabolic correction after administration of a double-stranded rAAV vector encoding human G6Pase. Another study showed a metabolic correction following rAAV8 injection in the retro-orbital sinus by the age of 2 to 4 weeks (5 × 10 12 to 3 × 10 13 vp/kg) for 90 weeks and, most importantly, showed that treated mice do not develop HCC, whereas all of the nontreated mice died. Treated mice showed a G6Pase activity of between 3% and 128% of normal 90 weeks after gene transfer. This study identified 3% G6Pase-alpha activity compared with the wild type as the threshold for phenotypic correction. Another study identified 2% activity as the threshold for HCC prevention in GSD1a, and Chou et al. identified 6% for GSD1b. But Brooks et al. showed that the majority of GSD1a-diseased dogs (four out of five) develop HCCs despite GT after long-term (4–8 years) follow-up, even though G6Pase activity was 21% to 52%.
Koeberl showed that transgene expression declines over time after successful AAV-mediated GT in young mice and dogs, which represents an additional challenge to the development of GTs for GSD1a. Landau et al. showed that this problem could be solved with the “targeted integration into a safe harbor under help of zinc finger nucleases” approach.
Another unsolved problem for clinical translation of GT for GSD1a is ongoing renal disease after liver-directed GT and for GSD1b, and also ongoing myeloid disease. Different studies show that rAAV8-based GT is not able to cure the renal disease of GSD1a, most likely because of the liver tropism of rAAV8. Some studies show that a switch to rAAV9 serotype could maybe improve kidney transduction, especially when delivered into the renal vein.
In summary, GSD1 is still a very challenging disease for GT because of the high risk of HCC in uncorrected hepatocytes and the different organs that have to be treated, especially kidneys and the myeloid system.
Primary Hyperoxaluria
Primary hyperoxaluria type 1 (PH1) is a rare inborn metabolic disease (1:100,000) characterized by alanine-glyoxylate aminotransferase deficiency, resulting in oxalate overproduction and oxaluria with the development of renal failure. The end stage of the disease is a condition called systemic oxalosis, which is life threatening. At present, curative therapy is preemptive LT or combined liver and kidney transplantation. PH1 is a very attractive disease for GT because the unmet medical need is high, but at the same time it is a challenging disease because treatment is necessary in small children, and transduction efficiency needs to be higher as in CNS, for example. The reason for this is ongoing oxalate overproduction from uncorrected hepatocytes, which is not compensated by corrected hepatocytes in this disease (cell-autonomous behavior). From clinical studies, it is known that replacement of one-third of the liver do not prevent kidney failure secondary to oxaluria. Salido et al. tested an rAAV5- and an rAAV8-mediated GT approach in a mouse model of this disease. Vectors were administered by single-tail vein injection with 5 × 10 11 to 1.5 × 10 13 vg/kg in 12- to 16-week-old mice. During a short follow-up of 50 days, the hepatic alanine-glyoxylate aminotransferase enzyme activity was 24.9% to 10.6%. A high dose of AAV8 and AAV5 results in oxaluria with no significant difference compared with the wild type, although AAV8 was superior to AAV5. Castello et al. showed, in their study, a successful phenotypic correction with a helper-dependent adenoviral vector approach. This study was performed in mice of similar age, like in Salidos’s study, but with a longer follow-up period (24 weeks). These studies show proof of concept, but major questions for clinical translation were not answered. First, especially in this disease, treatment early in life is necessary (renal damage caused is irreversible), and therefore, episomal rAAV GT is not suitable unless readministration becomes possible. Second, crucial transduction efficiencies have to be reached in humans; in these studies, 40% to 80% of hepatocytes have been transduced, but it is known that transduction efficiency of rAAV is lower in humans than in mice. Third, longer follow-up periods would be necessary to prove long-term persistence. This long-term persistence could be reached with the same approaches as mentioned for CNS, for example, preventing antibodies by rapamycin synthetic vaccine particles (SVP) to enable readmission. This approach could enhance transduction efficiency at the same time because transduction of rAAV is mainly driven by autophagy. Another approach described by Meliani et al. is to envelop rAAV vectors in exosomes. These exo-AAVs are less susceptible to antibody-mediated neutralization, and they enhance transduction efficacy, in this study from 20% to 40%. Even though targeted integration could be a solution for avoiding loss of transgene in the growing liver, this approach will have a low transduction efficacy for curing PH1 because corrected hepatocytes have no selection advantage and the disease behaves cell autonomous. That means that corrected cells can not prevent or reduce production of toxic metabolites from uncorrected cells. For all these new approaches, no studies with PH1 animal models exist so far.
Progressive Familial Intrahepatic Cholestasis
The disease group of progressive familial intrahepatic cholestasis (PFIC) is a group of disorders with intrahepatic cholestasis caused by different inherited defects. Except for PFIC3 (MDR3 defect), all of them have a normal gamma-glutamyl-transferase despite cholestasis. PFIC is caused by a defect of FIC1, mainly expressed in the liver and intestines. PFIC2 is caused by dysfunctional or missing bile salt export protein located only in the liver. In the last years, PFIC4, -5, and -6 caused by defects of tight junction protein 2, farnesoid X receptor, and Myosin VB (MYO5B) have been detected. So far, GT studies for these diseases are rare. Siew et al. presented a study about a hybrid AAV piggyBac transposon vector for GT in neonatal multidrug receptor 3–deficient mice at the European Society for Paediatric Gastroenterology Hepatology and Nutrition (ESPGHAN) Annual General Meeting 2014. They used a piggyBac transposon, on one hand, to overcome the loss of episomal transgene cDNA and, on the other hand, to overcome the low transduction efficiency of transposons. With a vector dose of 5 × 10 11 vg, they were able to prevent the development of liver fibrosis. For PFIC2, -3, and -4, GT will always have to deal with the remaining risk of HCC in uncorrected hepatocytes. PFIC1 has a significant unmet need, because LT in these patients often causes severe problems attributed to the remaining uncorrected defect of FIC1 outside the liver, especially in the intestine. But on the other hand, this implicates that GT would have to transduce these organs also, which is challenging.
Alpha-1-Antitrypsin Deficiency
Alpha-1-antitrypsin (AAT) deficiency is an inherited disease with a defect location in the liver. The defect AAT causes liver and lung disease. Protein misfolding leads to accumulation of AAT in the endoplasmic reticulum of hepatocytes and causes chronic liver injury, liver fibrosis, and HCC, and the aspect of missing AAT in circulation leads to lung emphysema because of missing antiprotease function. This is the reason why GT for this disease is challenging. One needs either a very high rate of gene correction to ameliorate the liver disease, which still has a remaining risk for HCC; or one needs a dual therapy: a transgene producing AAT for the lung and inhibition of mutant AAT expression in the liver. Li et al. successfully studied such an approach in the mouse model. Further targeted integration approaches are attractive for this disease because corrected hepatocytes will have a selection advantage and will, over time, replace the majority of uncorrected cells, but there is still a remaining risk for HCC.
Song et al. showed a proof of concept for CRISPR- and rAAV-mediated AAT correction, but they were able show only partially restored AAT serum levels and did not evaluate still-ongoing liver disease.
Some studies, like the one from Bjursell et al., focused on the disruption of the mutated gene to cure liver disease. Bjursell et al. used an adenovirus plus CRISP/Cas9 approach for this.
Multiple AAT deficiency studies in patients were conducted. These studies used an AAT transgene with a rAAV1 vector for intramuscular application. The study from Mueller et al. could only achieve 2.5% to 3% of the purported therapeutic level, but this could be shown to be stable over 5 years with some evidence that this low expression could have a clinical impact already. Of course, these approaches do not influence liver disease and risk of transgene antibody development because transgene is not produced in the liver. In contrast, clinical phase I studies are running with small interfering RNA to block the mutant AAT, which could cure the liver disease but has no influence on the pulmonary disease.
Ornithine Transcarbamylase Deficiency
Ornithine transcarbamylase deficiency (OTC) is the most common inherited disorder of urea cycle disorders (UCDs) in humans and causes hyperammonemia. The OTC gene is X chromosome linked and expresses the enzyme activity predominantly in the liver. In addition, heterozygous females may experience life-threatening elevations of ammonia in the blood and brain, leading to irreversible cognitive impairment and death. Available treatment focuses on carefully monitored, lifelong dietary protein restriction combined with alternate pathway therapy using sodium phenylbutyrate. So far, correction of the enzyme deficiency is only possible with LT.
From the beginning of GT, OTC has been one target disease. The first phenotypic correction by adenovirus-mediated gene transfer in the OTC mouse model (spf and spf ash ) was described in 1996. The following clinical study with adenovirus ended fatally for the treated patient and was an enormous setback for GT.
In 2006, an OTC animal study from Bell et al. raised concerns about rAAV and insertional mutagenesis. But at this point, it is important to mention that the mouse model used is known for higher risk for liver tumors and that only the rAAV LacZ vector–treated mice and not the rAAV OTC–treated ones have had a higher proportion of liver tumors. In 2012 to 2013, Alexander and Cunningham et al. showed in the adult spf ash mouse that after intraperitoneal injection of AAV8 vector encoding liver-specific promoter and murine OTC cDNA, 60% OTC activity was established. Cunningham et al., however, showed that this approach is not sufficient to maintain stable transgene expression for preventing hyperammonemia under short hairpin RNA exposure into adulthood when mice were injected as newborns. Because of a wide spectrum of clinical phenotypes in OTC with high mortality and morbidity in the first 6 months of life, LT may be indicated in infancy. Therefore, a potential alternative therapy should also be applicable in early life.
A recent study from Wang et al. showed sufficient GT with self-complementary rAAV8 in 2-month-old mice (heterozygote OTC mice, heterozygote female mouse model) until 18 months. Wang et al. performed a similar study in the classical OTC mouse model, the spf ash mouse. They injected adult mouse with their codon-optimized self-complementary rAAV2/8 vector of 3 × 10 11 and 1 × 10 11 genome copies and followed them for 56 days. All showed metabolic correction for this time; the high-dose group showed 68% enzyme activity compared with the wild type at day 56 after injection.
Another very important study in the field of GT was conducted on the spf ash mouse by Yang et al. in 2016. They could show a remarkable success for the Cas9- and rAAV8-mediated gene repair approach in newborn spf ash mice with a follow-up of 8 weeks and a high-protein diet in week 7. They found an HDR rate of 10% and an OTC activity of 20%. Very surprising, and maybe also important for other diseases, was the fact that the same therapy in 8- to 10-week-old mice resulted in aggravation of the disease because of large deletions on the OTC gene, which erased residual OTC activity in diseased mice. The number of correct HDRs was very low in these mice (0.3%–1.7%). This is a very important safety issue, which has to be taken into account in other GT trials with nucleases.
A further important aspect for GT in UCDs is the zoning of metabolic pathways within the hepatocyte because this can influence the efficiency of GT. For urea cycle defects, even a high number of transgene expressions can have low reduction of toxic products in animal studies, because rAAV8 produces transgene expression predominantly in pericentral hepatocytes in mice and dogs, but the urea cycle pathway takes place mostly in periportal hepatocytes. However, in nonhuman primates, rAAV8 shows transgene expression mostly in periportal hepatocytes. Therefore in diseases with metabolic zonation in the liver (for example, UCDs), it is difficult to extrapolate vector doses for human translation from studies in mice.
Tyrosinemia Type 1
Tyrosinemia type 1 (HT1) is an autosomal recessive disease based on fumarylacetoacetate hydrolase leading to abnormal tyrosine degradation and the accumulation of toxic metabolites. Although the tyrosinemia mouse model is a suitable model for GT studies because the phenotype is clear and corrected hepatocytes have a selection advantage, it is not a suitable disease for translational clinical studies because medical management with nitisinone [2-(2-nitro-4-fluoromethylbenzoyl)-1,3-cyclohexanedione] has revolutionized the prognosis for affected patients. Treatment cannot completely abolish the risk of hepatocarcinogenesis; however, this is also a main obstacle for GT in tyrosinemia, because uncorrected cells have a high risk of developing HCCs.
Paulk et al. showed that an AAV-mediated gene correction approach is feasible in vivo and can functionally correct the disease in both adults and neonatal mice with a point mutation on the Fah gene. rAAV8- Fah vectors with homologous sequences for HDR were injected into 3-day-old and 8- to 12-week-old mice intravenously at 1 to 2 × 10 11 vg/mouse. This is an important result because it showed that gene correction with HDR is feasible also without nucleases. The study by Junge et al. went one step further. They showed that even gene addition via HDR into the ROSA26 locus for the same disease but in another mouse model (FAH Δexon5 ) is sufficient to create long-term phenotypic correction without nucleases. However, both studies benefit from the fact that in HT1, corrected hepatocytes have an enormous selection advantage, but this is not the case in many other LBMDs, and further, this also implies that residual uncorrected cells have a high risk of developing HCCs. Therefore translation from this study to clinical studies is questionable. More animal studies on HT1 exist without adding significant new aspects or progress to translational aspects. For example, Shao et al. showed gene repair with adenovirus and Cas9 nickase in a rat model, and Pankowicz et al. applied an approach of metabolic reprogramming by blocking under the help of CRISPR/Cas9, an enzyme one step before fumarylacetoacetate hydrolase to render the phenotype benign. Furthermore, Hickey et al. (in pigs with lentiviral vector) and Van Lith (in mice with rAAV and CRISPR/Cas9) performed studies with successful ex vivo GT.
Wilson Disease
Wilson disease (WD) has a low unmet need for GT because effective medical treatment is available, and in patients with difficult neurological disease or liver cirrhosis at diagnosis, the GT would come too late, like conventional treatment. Otherwise, WD is a suitable disease for GT because corrected hepatocytes have selection advantages, but the risk for HCC in residual uncorrected hepatocytes is not as high as in tyrosinemia, for example, and treatment must not be done in first years of life. One assumes that around 40% of corrected hepatocytes are necessary to correct the phenotype.
There is only one recent study on GT in WD. Murillo et al. showed in a mouse model (6 weeks of age) that rAAV8 (1.5 × 10 12 vg/kg) gene addition can reach transduction efficiency, which can cure the phenotype of WD. Further analysis with this approach could even show that GT is capable of reducing the overall cerebral copper content.
Current Challenges of In Vivo Gene Transfer With Adeno-Associated Virus Vectors
Despite the small size of GT clinical trials for rare diseases and the limited number of studies conducted so far, the experience with liver gene transfer with AAV in humans has resulted in important knowledge on the safety and efficacy of the approach, allowing testing of strategies to achieve the goal of safe and long-term correction for a number of genetic and metabolic diseases with liver gene transfer.
Despite the evidence of long-lasting efficacy in adults, a number of issues remain to be addressed to fully exploit the potential of therapeutic liver gene transfer in all patient populations ( Fig. 33.7 ). Particularly for, but not limited to, liver gene transfer, these include:
- 1.
AAV vector and transgene immunogenicity;
- 2.
Genotoxicity risk associated with AAV gene transfer to the liver;
- 3.
AAV gene transfer persistence in a developing versus adult liver.
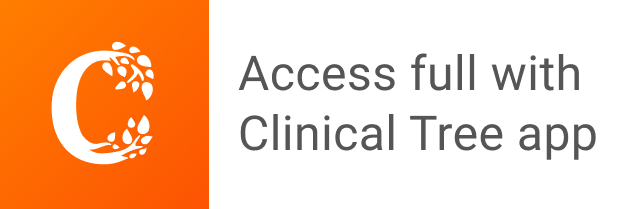