Abstract
The indigenous human microbiota is extremely diverse. New estimates of species richness, based upon culture-independent molecular techniques, put the total number of bacterial and archaeal “species” in the human gut at over 15,000. A delicately balanced symbiotic relationship between human host and microbial community exists. Natural selection, acting on host and microbiota, and the complex ecological interactions between the microbes help to shape the structure and function of the microbial community. There are numerous examples in the biomedical research literature demonstrating that an understanding of the principles that govern the structure and function of the microbial community has important implications for understanding health and disease. New high-throughput nucleic acid sequencing technology has allowed for much deeper probing of the microbial community. Studies of the microbiome are not only identifying community structure and membership, but are also revealing “what” the microbes are doing through examination of gene expression, protein products, and metabolites. We are just beginning to appreciate the importance of the human microbiome in health and disease and how we can manipulate it do “good” and promote health.
Keywords
Microbiota, Bacteria, Microbiome, Microbial ecology, Diversity, Richness, Evenness, Colonization, Metabolic niches, Metagenomics, Probiotics, Prebiotics, Antibiotics, Metabolites, Inflammatory bowel disease, Ulcerative colitis, Crohn’s disease, Obesity and colorectal cancer
32.1
Introduction
The human gut is one of the most diverse and rich ecosystems on Earth. It has been estimated that each person is home to over 100 trillion (10 14 ) bacterial and archaeal cells. This number is impressive when you consider that the sum total of all human cells in an individual person is approximately 10 trillion (10 13 ). The highest bacterial and archaeal densities found in the gastrointestinal tract (GIT) have been reported in the colon, where they approach 10 11 cells/mL. For comparison, the oceans and forest soils have estimated bacterial densities of 10 5 cells/mL and 10 7 cells/g, respectively. Thus, the GIT of a healthy human harbors one of the highest densities of bacteria on the planet, and this microbial consortium provides many benefits to its host.
Early estimates of the total number of bacterial species found in the GIT was 400–500. These early estimations were based upon culture-dependent techniques, and it was assumed that 90% of all bacteria present could be cultured. However, new data are suggesting that only 30%–40% of bacteria found in the human GIT can be cultured. It is evident that the preponderance of bacteria present in the gut are represented by species that have not yet been cultivated and new estimates, based upon culture-independent techniques, place the total number of “species” or operational taxonomic units (OTUs) in the human GIT at 15,000.
The human microbiome has been defined as the “ecological community of commensal, symbiotic, and pathogenic microorganisms that literally share our body space,” a key component of this definition is that it is not only the microorganisms, but the host or environment they occupy. The microbiota includes helminths (e.g., Cestoidea, Trematoda, and Nematoda), fungi, protozoa, viruses, bacteria, and archaea. For this chapter, the focus will be on the bacterial and archaeal members of the microbiota. Across the human population, there is tremendous diversity in the microbiota of individuals and there are many ecological, physiological, immunological, and toxicological benefits of the microbiome for the human host ( Table 32.1 ). In this chapter, the ecology and function of the gut microbiota will be presented. Key concepts such as the composition of the microbiota, microbial ecology, diversity, richness, evenness, gut microbial succession and colonization, metabolic niches, mechanisms that control diversity, bacterial metabolites, and the role of the microbiota in health and disease will be discussed.
Benefits | Examples |
---|---|
• Effects on epithelial barrier function | Improve tight junction formation; increase transepithelial resistance; increase expression of MUC1, MUC2, and MUC3, prevent the epithelial adherence of pathogenic microbes |
• Nutritional and metabolic benefits | Short-chain fatty acid production, e.g., acetate, butyrate, and propionate; production of biotin (vitamin H) |
• Immune regulatory effects | Downregulation of IL-12 and IFN-γ; Upregulation of IL-10 |
• Decreased visceral hypersensitivity associated with irritable bowel syndrome (IBS) | Some probiotics have been shown to be associated with a lessening of IBS symptoms, e.g., Lactobacillus plantarum 299v and Bifidobacterium animalis DN-173 010 |
• Metabolism of carcinogenic substances | Amines, e.g., 2-amino-1-methyl-6-phenylimidazo [4,5-b] pyridine |
• Pathogen exclusion (colonization resistance) | Helicobacter pylori cannot colonize the stomach in the presence of Lactobacillus salivarius |
The role the human microbiome plays in health and disease is just starting to be understood, a few of the examples include obese individuals can have a markedly different microbiome than lean individuals ; patients with inflammatory bowel disease (IBD) have altered microbiota, and they may have changes in their gut microbiota that precede a diagnosis, particularly in Roseburia spp., which have been shown to be decreased in those already diagnosed with IBD and as such, the manipulation of the microbiota using antibiotics, probiotics, and prebiotics might be useful in treating IBD ; and infants and adults with Celiac disease have a higher abundance of certain bacterial species in their GIT, that is, Bacteroides , Clostridium , and Staphylococcus , identified from the feces. These observations and many others have been the motivating force underpinning the National Institutes of Health (NIH) Human Microbiome Project (NIH HMP).
The NIH HMP roadmap for biomedical research has three main goals: (1) utilize new high-throughput screening technology to characterize the microbiome more completely by studying multiple body sites from 250 “normal” individuals; (2) determine if there are associations between changes in the microbiome and health and disease; and (3) standardize data resources and new technologies for the wider scientific community. Phase one of this project started in 2008 and landed two seminal papers in 2012, which examined a healthy or “normal” adult cohort. The collective findings from these papers were that (1) each body region was unique in its microbial community and (2) metabolic pathways were constant regardless of taxonomic composition. Furthermore, data from the HMP and other human microbiota studies can be added to epidemiological studies to better understand population health and human disease. Phase two of this project has begun, and using a multiomics approach (described later in this chapter), it aims to examine changes in three microbiome-associated conditions: (1) preterm birth, (2) IBD, and (3) type 2 diabetes.
To broaden the impact of microbiome research, in 2016, the White House Office of Science and Technology Policy announced a new National Microbiome Initiative (NMI). The goal of this project is to study different ecosystems, examining microbiome behavior under various conditions, and explore how microbial systems could impart protection or restore normal function after perturbation.
32.2
Overview of Culture-Independent Molecular Techniques for Characterizing the Human Gut Microbiome
Over 30 years ago, C.R. Woese pioneered the use of ribosomal RNA (rRNA) sequence comparisons for reconstructing the evolutionary history of microbes. With the introduction of improved sequencing technology, N.R. Pace and others developed culture-independent, molecular tools for assessing the ecology of microorganisms. The number of microbial phyla increased from Woese’s description of a dozen bacterial lineages to more than 100 major phyla; most of which do not include cultured representatives. Sequence analysis of polymerase chain reaction (PCR) amplicons for 16S rRNA gene genes has become the “gold standard” for assessing species richness in microbial communities.
The majority of culture-independent techniques are based upon the PCR amplification of the highly conserved 16S rRNA gene, which is a particularly useful molecular marker for identifying bacterial species. It is transmitted vertically without lateral gene transfer, highly conserved and not under natural selection. However, despite being relatively small (approximately 1.5 Kbp), it does have nine hypervariable regions that are useful for reconstructing evolutionary history (phylogeny). Conserved sequences allow for the design of broad bacterial kingdom primers that can create amplicons of individual 16S rRNA genes in a population. Differences in the numbers and sequences of 16S rRNA amplicons can be compared to identify and profile the myriad members of the human gut microbiome. Current molecular techniques can create microbial community fingerprints, locate and quantify bacteria geographically within the GIT, quantify and estimate relative bacterial abundance, and identify the members of the community. Also, with the advent of new high-throughput sequencing platforms, it is possible to perform metagenomic analysis of the entire community accurately, quickly, and relatively inexpensively.
Fluorescent in situ hybridization (FISH) is a technique that can be used to visualize and quantify bacteria abundance in their particular environments within the GIT. FISH uses fluorescent oligonucleotide probes that bind to a specific target sequence, that is, 16S rRNA, within the bacterial cell. Once the fluorescently labeled probe has hybridized with the target gene, a fluorescent microscope can be used to visualize the bacteria cells, allowing for the direct examination of spatial distribution and quantification. These techniques have also been adapted for flow cytometry, thereby allowing quantification of individual bacteria. Universal bacterial probes and probes for specific genera, for example, Bacteroides , Bifidobacterium , Streptococcus , Lactobacillus , and Clostridium , are available. It has been estimated that 90% of fecal bacteria can labeled by some type of taxon-specific FISH probes.
Even more widely used is the quantification of bacterial abundance using real-time quantitative PCR (Q-PCR). The primers for this assay can be kingdom-specific, phylum- specific, and even genus-specific. Targets for Q-PCR range from 16S rRNA or other highly conserved genes to taxon-specific gene targets. The data can be expressed (and normalized) as total bacterial gene copy number per unit sample or per single copy host gene, if biopsy or other tissue samples are analyzed.
Perhaps the most commonly used technique to determine community structure of the microbiota is to employ high-throughput sequencing techniques. Briefly, this approach requires the extraction and purification of genetic material, the preparation of a 16S-rRNA encoding gene amplicon library designed around the question or hypothesis being examined, sequencing of the material, and processing and analysis of the data. The Illumina platform is currently the platform of choice as it gives high-quality reads, is cost-effective (more sequence data per dollar), it generates longer sequence reads, and importantly, it supports data obtained on other platforms.
More recently, techniques, such as metagenomics, have been employed to study the functional role of gut microbe communities. This technique uses random sequencing of all the DNA extracted from a sample to predict polypeptides, which essentially catalogs all the genes of a given community without needing to identify individual community members. Metagenomics will be described in the context of health and disease later on in this chapter. However, a considerable portion (nearly half) of the microbial metagenome cannot be mapped to previously isolated genomes or even to annotated genes. A combined approach called meta-omics or multiomics circumvents this shortcoming by applying metagenomics, metatranscriptomics, metabolomics, and computational approaches to examine these functionally uncategorized microbial products such as proteins and metabolites. Fortunately, analytical computational tools such as Quantitative Insights Into Microbial Ecology (QIIME), the software package mothur, the web application METAREP, and others exist to help sort and make sense of data generated using these varied approaches.
Historically, other techniques were used to determine community structure and diversity. Commonly used techniques included denaturing and temperature gradient gel electrophoresis (DGGE and TGGE) and terminal restriction fragment length polymorphisms of the 16S rRNA gene (T-RFLP). Chromatograms showing the relative abundance of the individual fragments (T-RF’s) could then be constructed, and changes in the community were detected by the loss or gain of T-RFs. Additionally, constructing clone libraries of the 16S rRNA gene has been a useful method for community membership identification. Sequences can be compared using either classifier-based or OTU-based algorithms, to existing taxonomic databases, for example, the Ribosomal Database Project (RDP, http://rdp.cme.msu.edu/ ). While components of these previously used methods are still relevant, more precise and sophisticated techniques have been developed.
32.3
Applying the Principles of Microbial Ecology to Analyze the Human Gut Microbiome
Ecology is the study of the interplay between organisms, their physical environment, and other organisms that share the physical environment with them; hence, the study gut microbiome can draw many terms and analytical techniques from ecology. One of the key concepts in ecology is competition . Competition for resources, such as nutrients and space, can occur within a species or between species and is the major driving force behind natural selection and evolution. If one of the competitors is weaker or not as adept at utilizing a resource it will either die out or be excluded. Competitive exclusion is the end result of competition forcing an organism to be excluded from a habitat. In the case of gut microbes, this could be competition for the same nutrient, for example, carbon source. No two species will utilize the same resource with the same efficiency. Ultimately, one species will reach a higher population density and force the competitor out of that habitat. Resource partitioning or niche differentiation (a process of natural selection that will force competitors to use resources differently) is a way to avoid competition between species and allow for coexistence. The inhabitants of the GIT microbial community are adept at resource partitioning.
Another important ecological term is niche . An organism’s niche is not so much its physical placement within an ecosystem but its role in the ecosystem, often a metabolic role. No two species can ever occupy the same niche; competition will force one of the organisms out. Where and how bacteria get energy leads to the development of metabolic niches, for example, methane production, sulfate reduction, or carbohydrate fermentation.
Symbiosis is defined as the “living together” of two different organisms. Organisms can live with each other, on each other or within each other. The member within the relationship that has the other organism living in it or on it is the host . The member that is living in or on the host is the symbiont . If both members of the symbiotic union benefit from the relationship, it is termed mutualism . If one member benefits and the other is unaffected, it is called commensalism . The biomedical literature usually describes the members of the gut bacterial microbiome as commensals; however, we now understand much more about the complex relationship between humans and their microbiome and this symbiosis is clearly mutualistic. Iťs been suggested that because the health of humans is so intertwined with their microbiome (and vice-versa), that we are a superorganism. For example, the microbiota contains within its genome metabolic pathways that humans do not possess but that are necessary for our survival. Much of the microbiota cannot thrive outside of the human host. Given the extent of codependence between host and symbiont it seems fair to consider the idea of superorganism. Pathogenic species can be considered “cheaters” that benefit from the existing microbial community and at the same time can negatively impact the fitness of the host and microbial community.
Lastly, ecological succession is the process of changes in species structure within an ecological community. New habitats are first colonized by a pioneer species —this is autogenic succession . Pioneer species are able to withstand more extreme or less favorable living conditions. Their presence in the environment can change both biotic and abiotic components of that environment and over time new species that would not have normally been able to colonize that habitat can do so. If an existing habitat is perturbed (fire, flood, act of god, or antibiotics in the case of microbes), the succession that follows is called allogenic . Succession will be an important concept for understanding colonization of the gut by microbes. The indigenous microbiota that has taken up residence within our GIT is called autochthonous . Microbes that are not part of our resident microbiome and are transient in nature are called allochthonous . The allochthonous members may come from diet, water, or from other sources in our environment.
There are several ways to measure the membership and structure of a microbial community. First, a community is a group of different individual species that interact with each other in the same place at the same time. The most basic measure of a community is species richness . Richness is the total number of species present. Abundance is a measure of how many individuals of each species there are. With species richness and abundance, the species evenness can be calculated. Evenness is a measurement of individual variation in the abundance of the species present. For example: two communities exist (Com 1 and Com 2). Com1 has three species all with an abundance of 10. This community would be considered very even. That is, you have equal numbers of individuals for all three species ( n = 10). In Com 2, you also have three species; however, one species has an abundance of 25 while the remaining two have an abundance of 5. Both Com 1 and Com 2 have the same species richness ( n = 3), but the evenness is quite different. Com 2 two would have lower evenness because it is dominated by individuals of one species. With richness and evenness, you can calculate species diversity . Diversity measures the corresponding variation in richness and evenness. Communities with higher evenness are usually more diverse. In classical ecology, there are myriad ways to calculate diversity. Some of these diversity metrics, for example, Shannon’s Index or Simpson’s Index have been commonly used to describe the diversity of host-associated microbiota. The measurement of membership, richness, evenness, and diversity within a community is called the alpha diversity . Comparing the diversity between two different communities is called the beta diversity . Beta diversity measurements compare how similar or dissimilar the communities are based on changes in membership and structure, for example, Jaccard’s Coefficient of Community Similarity, Sorenson’s Similarity Index, Bray-Curtis Distance, and Morisita-Horn Index. However, one drawback to diversity calculations is that low abundance taxa are frequently not sufficiently quantified. One approach to better estimate diversity in low abundance taxa is to calculate the Tail statistic ( τ ) based on 16S data. Additionally, factors such as stability are being examined. It has been long suggested that healthy microbiomes are stable, and that although between individuals the microbes might be different, their individual microbiomes tend to keep the same key species over time. Recently, a theory to study stability has been proposed; this theory aims to identify key principles that underlie stability such as (1) the probability a community will return to its previous state after small perturbations, (2) the population dynamics during the return, and (3) how long the return takes. Altogether, these types of analyses facilitate discrete measurements and comparisons of highly complex microbial communities among sites and individuals.
In addition to taxonomic approaches, microbes can be analyzed at a molecular level using an OTU-based approach. An OTU can be defined as a collection of 16S rRNA sequences that have a certain percentage of sequence divergence. For example, as a rule of thumb for full-length 16S sequences, two OTUs with ~ 3% sequence divergence or ~ 97% similarity are often considered the equivalent of different “species.” At higher taxonomic levels, higher levels of sequence divergence can be used: genus (~ 5%); family/class (~ 10%); and phylum (~ 20%). It is important to understand how an OTU is created and how richness is estimated. Creating OTUs from raw sequence data can be a computational challenge; however, programs have been developed and are constantly being refined that can generate OTUs from large datasets and perform diversity and statistical analyses. One example is the previously mentioned open-source software program, mothur , which can be used to filter, trim, align, remove chimeras and group 16S rRNA sequences into OTUs. Several hundred thousand DNA sequences can be aligned and binned into OTUs using mothur in several hours.
With any community data, there will always be limitations to any sampling technique. Practically speaking, the total species richness is a function of sampling effort and no microbial community has ever been exhaustively sampled. However, there are methods available for estimating the species richness of microbial communities, for example, Chao1 estimator of species richness, which will utilize richness data, gathered for a particular community. Chao1 is based upon the idea that rarer species will carry the most information about the missing species. Chao1, therefore, places emphasis on those members that occur only once or twice in a community survey when calculating the estimate of species richness. In summary, the mathematical tools developed and utilized by environmental microbial ecologists are instrumental for studying the complex community of the human microbiome during health, perturbation, and disease.
32.4
Membership and Diversity of the Human Gut Microbiome
The indigenous human microbiome is dominated by two bacterial phyla: Firmicutes and Bacteroidetes. In many studies, the Firmicutes and Bacteroidetes account for greater than 98% of the bacteria present in the human gut ( Fig. 32.1 ). A total of nine bacterial divisions have been reported from the human gut, that is, Firmicutes, Bacteroidetes, Actinobacteria, Fusobacteria, Proteobacteria, Verrucomicrobia, Cyanobacteria, Spirochaetes, and VadinBE97. Archaea are also present in the human gut. Altogether, the human gut microbiome is extremely diverse and has been estimated that 15,000 “species” (defined as 3% OTUs) may reside within the human gut ( Fig. 32.2 ), supplanting earlier estimates, based upon culture-dependent techniques, of 400–500 species.


The density and diversity of bacteria increase distally from the stomach to the colon ( Table 32.2 ). The membership of bacteria present in the various regions of the GIT is also different. The stomach is less diverse than any of the regions of the small intestine, which in turn are each less diverse than the colon. The low pH of the gastric environment plays an important role in shaping that microbial community. Examples of bacteria that have been successfully cultivated from the stomach are lactobacilli, Bacteroides spp., Haemophilus spp., Helicobacter spp., Micrococcus spp., Neisseria spp., Streptococcus spp., Staphylococcus spp., and Veillonella spp. Culture-independent techniques have identified 128 distinct phylotypes from the stomach.
Organ | Density (CFU/mL) |
---|---|
Stomach | 10 1 –10 3 |
Duodenum | 10 1 –10 3 |
Jejunum/ileum | 10 4 –10 7 |
Colon | 10 11 –10 12 |
The small intestine is more diverse than the stomach; however, diversity, membership, and levels change along the length of the small intestine. Increased peristalsis of the duodenum is a factor that shapes the microbial community gradient of the GIT region. Common bacterial inhabitants of the duodenum are Streptococcus spp., Lactobacillus spp., Bacteroides spp., Bifidobacterium spp., Staphylococcus spp., and the Enterobacteriaceae. The microbial communities of the jejunum and ileum are more diverse than the duodenum and are made up of both facultative anaerobes and anaerobes. However, as transit time slows in the ileum the microbial community membership begins to switch to increasing numbers of anaerobic species. The microbial community of the cecum and colon is more complex than the jejunum and ileum and becomes dominated by anaerobes, for example, Firmicutes and Bacteroidetes make up the majority membership of the colon. The vast majority (97%) of bacteria present in the entire GIT are strict anaerobes while 3% are facultative anaerobes.
32.5
Colonization and Succession of the Human Gut Microbiome
Previously, the prevailing thought was that at birth the infant GIT was completely sterile; however, this has become controversial. It has recently been suggested that various components of the fetal environment (placenta, amniotic fluid, cord blood, etc.) may contain microorganisms. Indeed, meconium, the first bowel movement from newborns, contains bacterial communities. On the other hand, others have found that between the potential low bacterial load and the possibility of contamination during sample preparation, there is no strong evidence for a placental microbiome. Regardless, the Firmicutes and Bacteroidetes that come to dominate the adult GIT cannot grow readily outside of their human host. How then does the gut become colonized by the rich and diverse community that is found in adults? What are the effects of delivery methods, antibiotics, and diet on initial colonization and succession? These questions, which are critical for human health, rely on many of the ecological principles that have been studied by macro and microbial ecologists.
A better understanding of how the bacterial community forms (pioneering species), how community membership naturally changes over time (succession), and functional redundancy (resource partitioning) will be critical for understanding the role of the microbiota in health and disease. The human GIT is first colonized by facultative anaerobes such as Escherichia coli and Enterococcus spp. These early colonizers or “pioneers” are essential for transforming the environment into one that can be successfully colonized by other species. The early neonate GIT is not suitable for colonization by the majority of the microbes that will come to inhabit that ecosystem. The pioneering facultative anaerobes consume oxygen, produce carbon dioxide, alter the pH, provide additional sites for adhesion, produce nutrients, and lower the redox potential making the environment suitable for strict anaerobes that will come to dominate the microbial community. By the first 2 weeks of life, obligate anaerobes begin to appear ( Bacteroides spp. and Bifidobacteria spp.).
The maternally derived pioneering species, and those species that follow, are very important in shaping the microbial community of the GIT. However, there are many other factors, besides microbial inheritance, that shape the community. Selection pressures on the host and the chemical and physical environment play crucial roles in community formation. Natural selection, acting on the host, impacts microbial community membership and structure. This form of selection is called “top-down” selection and favors stability and functional redundancy.
Research suggests that the neonate gut microbial community is inherited from contact with the feces and vagina of their mothers as well as the immediate environment. It was widely assumed that the first bacteria to colonize the neonate gut arose from the maternal vagina. However, it seems that during early neonate ontogeny, the bacteria that are most successful at colonizing the human gut come from contact with the maternal fecal microbiota. However, the microbial community of the infant and adult GIT is different. The adult microbial community is more stable, while the developing infant microbial community fluctuates temporally ( Fig. 32.3 ). Fig. 32.3 shows within-individual fluctuations of certain taxonomic groups, and it shows the differences in abundance between individuals. In nearly all cases, the early microbial community was shown to be dominated by gamma-proteobacteria, while others meta-studies have shown that both proteobacter and Bacteroidetes colonize the early gut. By 36 weeks of life, infants born vaginally, begin to have a microbial community that resembles the adult gut and by approximately 2 years of age the adult microbial community is established. Ontogenetic change in community stability and structure is attributed to the incorporation of solid foods in the diet. Infants born via caesarian have an altered GIT colonization compared to those born vaginally, although it appears to recover after about 6 months.

Functional redundancy is the “ability of one microbial taxon to carry out a process at the same rate as another under the same environmental conditions.” Within the gut microbial community, there are functional groups (guilds) of bacteria and Archaea. That is, there are many species of bacteria and Archaea that can perform the same function, for example, fermentation, methane production, and sulfate reduction. Functional redundancy is what drives resource partitioning in the microbial community. If microbes can coexist by “sharing” resources, this can alleviate or reduce competition. As stated earlier in this chapter, competitive exclusion is a result of competition. If two species occupy the same niche, then competition for that resource can drive one species to local extinction. Functional redundancy and resource partitioning can increase the stability of the gut microbial community by making it more diverse. The more diverse the ecosystem the more likely it is to withstand perturbations and repel invaders. A diverse and robust GIT microbial community can prevent pathogens from colonizing. Selection pressures on the microbe via the host and microbe-microbe interactions is called “bottom-up” selection and this tends to drive microbes to become functionally specialized to avoid competition.
The microbial community of the gut can carry out key metabolic activities including fermentation, sulfate reduction, and methanogenesis. The majority of species, belonging to the Phyla Firmicutes and Bacteroidetes, are involved in fermentation. Undigested carbohydrates (resistant starches, plant polysaccharides, and oligosaccharides) constitute the majority of food available for fermenting bacteria. Two bacterial genera ( Desulfovibrio spp. and Desulfotomata spp.) have been identified as sulfate reducers. The Archaeal members that have been shown to produce methane are Methanobrevibacter smithii and Methanosphaera stadmaniae . Only members of the Archaea have been shown to produce methane. Bacteria involved in fermentation dominate the GIT microbial community because the vast majority of available nutrients are derived from host-ingested carbohydrates. Understanding the dynamic processes of pioneering species and community formation, succession, and resource partitioning will be critical for future research involved in microbiome manipulations.
32.6
Modulation of the Gut Microbiota
It is possible to modulate the human microbiome. That is, it can be changed by exogenous sources, for example, antibiotics, probiotics, prebiotics, and diet. Changes or alterations to the structure and membership of the microbial community, that is, dysbiosis, can have benefit to the host or it can cause harm. This type of ecosystem management will no doubt have an impact on the treatment of diseases and in maintaining the health of the host. Humans rely on their microbiota to act as a barrier between the host and potential pathogens and to help prevent the overgrowth of existing microbes that could cause harm. The gut-associated immune system and the GIT microbial community have evolved together to form a barrier/interface between the host and external world. The GIT microbial community has been shown to play a role in immune education, and perturbations to the microbial community have been implicated in the development of systemic and localized inflammatory disease. Changes in the microbial community have been linked to the development of vaginitis, atopic dermatitis, eczema, food allergy, IBD, and pouchitis. In order to manipulate the microbiome to do “good” and not “harm,” future research will need to unlock the complex and enigmatic community dynamics of the microbiome. Examples from both human and mouse GIT microbiome research demonstrate the complexity of understanding changes that occur in the microbial community structure and membership.
32.6.1
Antibiotic-Associated Dysbiosis
It has long been appreciated that different classes of antibiotics affect the human gut microbial community, both targeted and off-target. In a review examining the effects of antibiotics on gut microbiota, Lange et al. constructed a brief table describing the impacts of different microbial classes on the bacterial composition and their effects on gut immunity. The use of antibiotics can open niches that were otherwise occupied and allow for new species (good or bad) to take up residency. For example, changes in human GIT community structure after exposure to the fluroquinolone antibiotic, ciprofloxacin, has shown that much of the community is altered. Dethlefsen et al. reported that all aspects of the community, that is, diversity, richness, and evenness, were decreased and the abundance of approximately one-third of the species present was changed. The loss of diversity may cause acute human disease by impacting the role of the GIT microbial community on nutrition, metabolism, and pathogen resistance. After antibiotic treatment was stopped, many of the communities rebounded and closely resembled the original community. In some cases, it took as many as 6 months for the community to rebound. It has been suggested that broad-spectrum antibiotics, especially those that have activity against anaerobes, might cause longer lasting changes in the gut microbial community.
Antonopoulos et al. demonstrated a long term change in the gut microbiota in mice following treatment with the third-generation cephalosporin, cefoperozone. This study utilized a pyrosequencing-based approach to measure community dynamics in mice directly after treatment and in mice that were allowed to recover 2 for weeks post treatment. In control mice, the microbial community was dominated by the two phyla Firmicutes (74% of community) and Bacteroidetes (23%). The phylum Proteobacteria represented 1% of the community. However, mice exposed to cefoperazone for 10 days had a markedly different microbial community structure. In these mice, the dominant phylum shifted to the Proteobacteria (71%) and the Firmicutes and Bacteroidetes dropped to 7.5% and 16.8%, respectively. Interestingly, of the Proteobacteria found, the most abundant family was the Enterobacteriacaea (93%). Mice that were allowed to recover for 2 weeks regained most of the original microbial community structure. The Firmicutes, Bacteroidetes, and Proteobacteria represented 71%, 22%, and 5.7%, respectively, of the community.
Garner et al. demonstrated changes in the mouse intestinal microbiome during Salmonella enterica colonization of the mouse ileum after exposure to the antibiotic streptomycin. Changes in the microbial community were ascertained by creating 16S rRNA clone libraries and Q-PCR. C57BL/6 mice were treated with streptomycin, to disrupt the microbial community, and then inoculated with S. enterica . It was reported that untreated mice had an ileum microbial community composed of Firmicutes (82%) and Bacteroidetes (16%). The dominant Family identified from the Firmicutes was Clostridiaceae (34%). It was further reported that 32% of the Clostridiaceae were segmented filamentous bacteria (SFB). The SFB can provide protection against Salmonella colonization. Garner et al. reported a 10-fold decrease in the abundance of SFB and a significant increase in S. enterica in mice treated with streptomycin. It was concluded that streptomycin can alter the community structure and more importantly they identified changes at the population level; that is, SFB loss, can promote the invasion of, and successful colonization of the ileum by a pathogenic strain of bacteria. Antibiotics can disrupt microbial community structure; however, the community has the ability to rebound after treatment is stopped. However, compounding treatments of antibiotics can lead to what ecologists have termed “ecological surprises” where multiple rounds of antibiotics can result in incomplete recovery of the microbiome. Furthermore, antibiotic use can select for antibiotic resistant bacteria and the resulting dysbiosis can promote the horizontal transfer of resistance genes.
32.6.2
Modulatory Effects of Probiotics and Prebiotics
The idea of “probiotics” was originally put forth by the Russian scientist Eli Metchnikoff in the early 20th century. Metchnikoff suggested that lactobacilli could help promote GIT health by displacing toxin-producing bacteria. Since Metchnikoff, a probiotic has been formally defined by the FAO/WHO as “live microorganisms which when administered in adequate amounts confer a health benefit on the host.” The definition of probiotic has been expanded to include that (1) it cannot be nonpathogenic and nontoxic; (2) it must have a large number of viable cells; and (3) it is capable of surviving and metabolizing within the gut. Almost all of the current probiotics are allochthonous and do not permanently colonize the gut. If colonization does occur, it is only temporary; however, the data are lacking on whether adhesion occurs on the epithelium or mucus. As probiotics transit through the gut, they can have modulatory effects on the microbiota and confer health benefits. Examples of possible health benefits include (1) increased resistance to infectious diseases in the GIT; (2) suppression of cancer; (3) alleviate symptoms due to the malabsorption of lactose; (4) reduce serum cholesterol; (5) improve digestion; and (6) improved GIT immunity. Examples of bacterial probiotics include the Lactobacilli ( Lactobacillus acidophilus , L. casei , L. brevis , and L. reuteri ), Bifidobacteria ( Bifidobacterium bifidum , B. infantis , B. animalis , B. thermophilium , and B. longum ), and Gram-positive cocci ( Enterococcus faecium , Lactococcus lactis , and Streptococcus salivarius ).
The idea of “good” microbes out-competing “bad” microbes for resources and space in our GIT to promote health and discourage disease is an exciting and fascinating prospect. For example, the probiotic, Lactobacillus helveticus , has been shown to prevent E. coli O157:H7 from adhering to the intestinal epithelium. Without adherence to the epithelium, the pathogenesis of disease caused by E. coli O157:H7 is prevented. In another study, a significant increase in MUC2 (mucin) gene expression was shown to occur in the presence of the probiotic , L. acidophilus. The increased MUC2 expression significantly reduced the ability of E. coli O157:H7 to attach to epithelial cells. The probiotic, L. lactis , produces Lacticin 3147, a broad spectrum bacteriocin (bacterial toxin that can inhibit the growth of other bacteria), and has been shown, in vitro, to significantly reduce numbers of Clostridium difficile via the rapid lysis of bacterial cells. The bacteriocin, Acidocin 1B, produced by L. acidophilus GP1B, was shown, in vitro, to have significant antimicrobial effects on many gram-negative pathogenic bacteria, e.g., E. coli O157:H7, Pseudomonas aeruginosa , Shigellasonnei , Listeria monocytogenes , and Yersinia enterocolitica.
Kabir et al. orally administered L. salivarius to gnotobiotic BALB/c mice. One week after inoculation with L. salivarius , the pathogenic bacteria Helicobacter pylori was orally administered to the mice. Results from their data show that H. pylori could not colonize the stomach in the presence of L. salivarius . In the absence of L. salivarius , the H. pylori could colonize the gut and cause severe gastritis. Aiba et al. reported that L. salivarius was capable of producing large amounts of lactic acid in vitro and thus inhibiting the growth of H. pylori. These authors also showed that H. pylori was undetectable in mice inoculated with L. salivarius . They suggested that inhibitory compounds, for example, lactic acid, other volatile acids and bacteriocins, produced by L. salivarius , were responsible for inhibiting H. pylori growth. Further, it was shown that L. casei and L. acidophilus were not capable of producing large amounts of lactic acid and, thus, could not inhibit the growth of H. pylori .
In one study, treatment with the probiotic Lactobacillus GG improved symptoms in a number of individuals suffering from C. difficile- associated diarrhea and abdominal pain. In all cases, patients had been suffering from relapsing colitis due to C. difficile infection and had been treated with antibiotics but to no avail. The incidence of traveler’s diarrhea was significantly decreased in Egyptian tourists that were given a probiotic cocktail of L. acidophilus , L. bulgaricus , B. bifidum , and S. thermophilus. Probiotics have also been used to treat GIT ailments such as, infantile diarrhea, antibiotic associated diarrhea, and foodborne pathogens. There is evidence to support the idea that probiotics are capable of discouraging the growth and colonization of pathogenic microbes. Unfortunately, it has been difficult to identify a single probiotic that is effective in all individuals, which is likely due to differences in the microbiome between individuals and the need to match a specific strain of probiotic with a restricted range of bacterial community structures for optimal effect. On the other hand, it is also possible that the benefits of probiotics are not due to single strains, but to a community of strains that allow the reestablishment of homeostasis. While there are many commercially available products that strive to confer the benefits of probiotic bacteria to dysbiotic guts, the mechanism and strain or community specificity is not well understood.
The concept of prebiotic was originally proposed by Gibson and Roberfroid and was defined as a “nondigestible food ingredient that beneficially affects the host by selectively stimulating the growth and/or activity of one or a limited number of bacteria in the colon, and thus improves host health” although a more broad definition might include compounds that stimulate the “ecological biodiversity.” Prebiotics should also not be absorbed in the small intestine, they should not be fermented by oral bacteria, well fermented by “good” bacteria and poorly fermented by pathogenic bacteria. The majority of prebiotics are designed to promote the growth of lactic-acid producing bacteria (LAB). It is the LABs that have been shown to be the most promising in promoting health. By choosing the correct prebiotics it will be possible to selectively promote the growth of specific groups of bacteria. Examples of prebiotics include fructooligosaccharides, inulin, galactooligosaccharides, lactulose, lactitol, xylooligosaccharides, cereal fibers, resistant starches, lactosucrose, soybean oligosaccharides, isomaltooligosaccharides and lactitol.
Prebiotics, naturally found in human breast milk, that is, human milk oligosaccharides, are being added to baby formula to promote the growth of certain Bifidobacteria species. The prebiotic, lactulose, has been used to treat hepatic encephalopathy. Lactulose has also been used a laxative to treat constipation. A significant reduction of atopic dermatitis in infants was seen in patients given a prebiotic mixture of fructooligosaccharides and galactooligosaccharides. Clinical trials involving patients suffering from ulcerative colitis reported a reduction of inflammation, decreased proinflammatory cytokines, and an improvement in the regeneration of epithelial cells when given fructooligosaccharides and inulin. Inulin was shown to reduce mucosal inflammation, increase butyrate levels, and lower the gut pH in patients with pouchitis. It is possible to alter the physiology of the GIT microbial community by changing the food available for fermentation, resulting in the production of different short-chain fatty acids (SCFA); SCFA are byproducts of bacterial metabolism. For example, increasing consumption of resistant starch in healthy young adults results in changes in fecal butyrate concentrations, although these changes were heterogeneous likely due to the varied colonic microbiomes between individuals. A change in the type of SCFA produced, however, does not necessarily result in changes in microbial community structure. The right prebiotics can promote the production of healthy bacterial metabolites without compromising stability of the microbial community. The manipulation of the microbial community using probiotics and prebiotics has the potential to promote intestinal health while discouraging the colonization of bacterial pathogens.
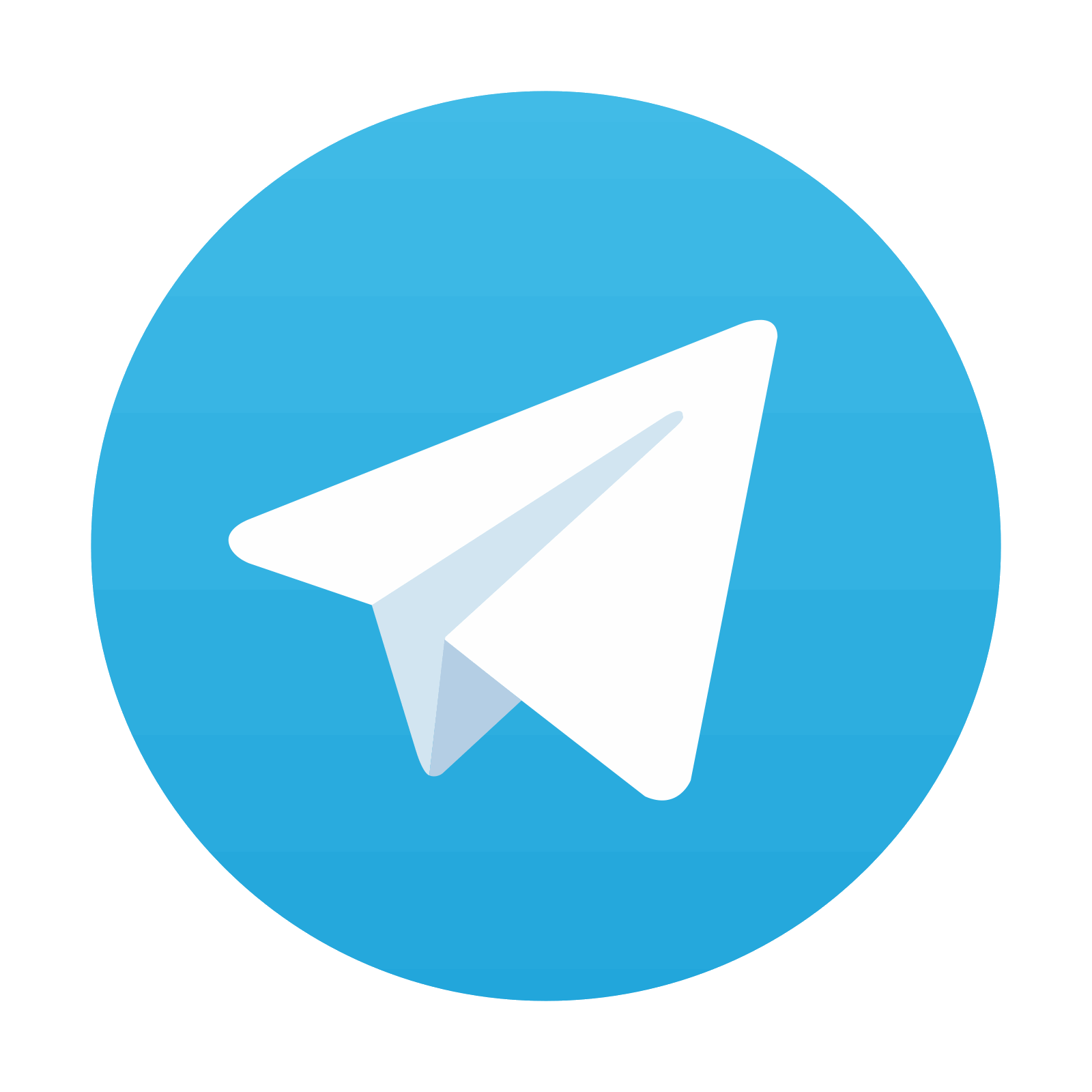
Stay updated, free articles. Join our Telegram channel
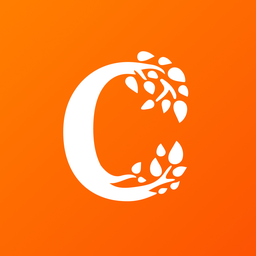
Full access? Get Clinical Tree
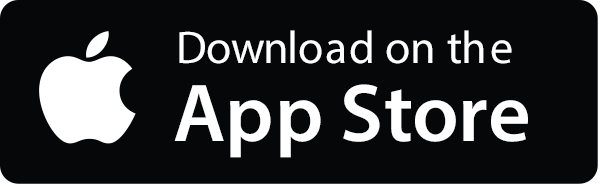
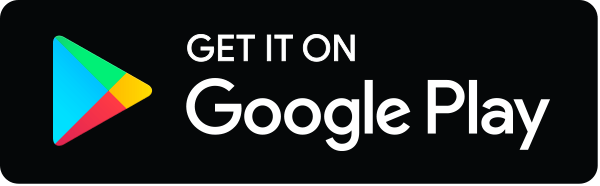