Fig. 5.1
Negative staining of urinary exosomes from normal human subjects using electron microscopy at 41,000× magnification. This magnification was used to show a variety of exosomes from a 200,000×g low-density pellet based on the 20–100 nm size and round, cup-like shape
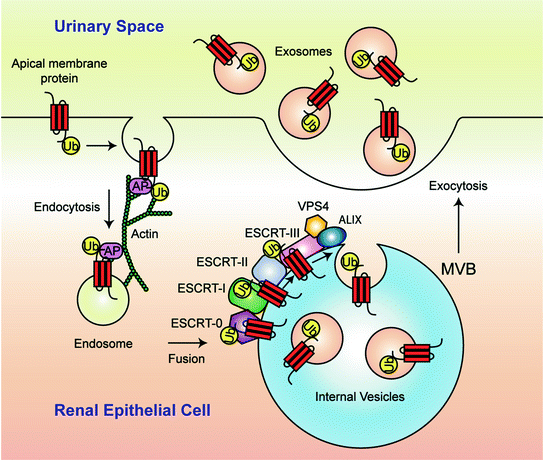
Fig. 5.2
A model for multivesicular body (MVB) formation and exosome secretion into urine. Monoubiquitination (Ub) is the signal that marks plasma-membrane proteins for incorporation into MVBs. Monoubiquitinated proteins are endocytosed by a process dependent on adaptor proteins (AP) and sorted in the endosomal pathway. Fusion with and internalization of the ubiquitinated cargo into the MVB requires assistance from endosomal sorting complexes required for transport (ESCRT)-protein machinery (ESCRT-0, ESCRT-I, ESCRT-II, and ESCRT-III), VPS4, and ALIX. Eventually the outer membrane of the MVB fuses with the apical plasma membrane of the renal epithelial cell, releasing exosomes into the urinary space
Based on these data, the authors concluded that low-density urinary vesicles are largely made up of exosomes derived from the internal vesicles of MVBs of every epithelial cell type facing the urinary space (Fig. 5.2). Importantly, urinary exosomes contain proteins that potentially reflect the physiological or pathophysiological state of their cells of origin, and therefore, exosome isolation, as highlighted by the authors, may provide an efficient first step in biomarker discovery in urine.
To expand their initial findings, in 2009, the same investigators conducted a large-scale proteomic study of human urinary exosomes using a more sensitive LC-MS/MS system. Overall, 1,132 proteins were identified including 14 phosphoproteins. The Online Mendelian Inheritance in Man (OMIM) database highlighted that 177 of these proteins were disease-related proteins, 34 of which were associated with renal diseases and/or hypertension [19]. An online database of human urinary exosomal proteins can be found at http://dir.nhlbi.nih.gov/papers/lkem/exosome/. These studies showed proof-of-principle that the proteomic analysis of urinary exosomes can serve as an excellent tool for discovering disease-related biomarkers.
In addition to a wide range of proteins [2, 19, 20], Miranda et al. [5] discovered in 2010 that nucleic acids, including mRNA and microRNA (miRNA), were contained and preserved in urinary exosomes. The identified mRNA species encoded proteins from all segments of the renal tubule. These studies demonstrated that exosomal genetic materials have potential roles for urine biomarker discovery and that exosomal RNAs may have functional roles, i.e., in cell-to-cell communication along the nephron [21].
5.3 Collection, Isolation, and Normalization of Urinary Exosomes
5.3.1 Collection and Storage of Urine Samples
For clinical and research purposes, it is important that urine destined for isolation of exosomes is collected and stored in a standardized way. There are three important steps that should be addressed to standardize the collection and storage of urine: (1) addition of protease inhibitors and preservative during urine collection, (2) storage of urine at stable temperatures, for short-term and long-term use, and (3) extensively vortexing urine samples after thawing [12, 22] (see more at http://www.niddk.nih.gov/research-funding/at-niddk/labs-branches/kidney-disease-branch/renal-diagnostics-therapeutics-unit/sample-collection-storage-exosome-analysis/Pages/default.aspx). Without the use of protease inhibitors, various key proteins often detected in urinary exosomes can degrade. The typical cocktail of protease inhibitors and preservative includes phenylmethanesulfonyl fluoride (PMSF), leupeptin, and sodium azide. From our own experiences, storage of urine at −80 °C provides a more stable condition than storing samples at −20 °C. With extensive vortexing after thawing, samples stored at −80 °C have the highest recovery of exosomes—up to 100 % when compared to freshly processed urine. In contrast, urine stored at −20 °C has only 87 % exosome recovery after extensive vortexing. Nanoparticle tracking analysis of urinary exosomes has recently confirmed a storage condition of −80 °C with the addition of protease inhibitors is the best [22]. Urine collected in the morning, both first and second urine of the day, has similar exosome contents and can be used interchangeably for experimental research purposes [12].
5.3.2 Processing Procedures for Isolation of Urinary Exosomes
After the discovery of potential renal disease-related biomarkers in urinary exosomes, researchers have worked to identify a variety of methods for faster and more efficient ways to collect urinary exosomes for clinical applications. This exploration began because the first method of isolation, ultracentrifugation, requires long processing times and access to expensive equipment. Despite the applications of novel techniques, including membrane filtration and exosome precipitation methods, ultracentrifugation methods show the highest exosome purity. Table 5.1 shows a comparison of urinary exosome isolation techniques.
Table 5.1
Comparison of urinary exosome isolation techniques
Methods | Advantages | Disadvantages | References | |
---|---|---|---|---|
Ultracentrifugation | Ultracentrifugation | • Reproducible results • High yield of intact proteins and nucleic acids | • 4 to 5 h to process single sample • Some contamination of highly abundant proteins • Expensive equipment | [2] |
Double-cushion ultracentrifugation | • Less contamination of highly abundant proteins • Reproducible results | • Long processing time • Tedious separation techniques • Expensive equipment | [23] | |
Sucrose gradient ultracentrifugation | [24] | |||
Ultracentrifugation—size exclusion chromatography | [25] | |||
Membrane Filtration | Nanomembrane filtration | • Shorter processing time, 0.5–2 h • Many samples can be processed at one time • Relatively inexpensive • Can be used in a clinical setting | • Possible clogging of membrane • Sample loss • Contamination of highly abundant proteins | [3] |
Micromembrane filtration | [26] | |||
Precipitation | Precipitation by ExoQuick-TC | • Shorter processing time, 0.5–2 h • Yields intact RNA • Relatively inexpensive • Can be used in a clinical setting | • Low purity of protein • Modified protocol | [27] |
Ultracentrifugation was the original method of reproducibly isolating urinary exosomes [2]. This technique requires collecting urine in the collection solution (protease inhibitors and sodium azide), spinning the urine at a low speed (17,000×g) to remove whole cells, large membrane fragments, and cellular debris, followed by spinning the supernatant at a high speed (200,000×g) for 1 h to pellet the exosomes (for more details please check http://www.niddk.nih.gov/research-funding/at-niddk/labs-branches/kidney-disease-branch/renal-diagnostics-therapeutics-unit/exosome-preparation/Pages/default.aspx). This initial low-density exosome pellet commonly includes some contamination of highly abundant urinary proteins, including albumin and uromodulin, also known as Tamm-Horsfall protein (THP) [2, 23]. THP forms double-helical fibrils by a disulfide cross-link zona pellucida (ZP) domain, which entraps exosomes and is co-isolated with the low-density pellet. Adding the reducing agent dithiothreitol (DTT) with a subsequent high-speed spin reduces the presence of THP in the low-density pellet [2]. However, this method does not completely decontaminate the sample of all THP and other abundant proteins. Alternatively, a more purified exosome pellet can be obtained using an additional step of double-cushion ultracentrifugation [23] or the use of heavy water and a sucrose gradient in ultracentrifugation [24] to separate the exosomes and contaminating proteins based on density, whereas ultracentrifugation followed by size exclusion chromatography (UC-SEC) can be used to separate contaminating proteins from exosomes based on molecular weight [25]. However, these methods are still time-consuming and labor intensive and require expensive equipment.
In 2007, Cheruvanky et al. [3] used commercially available nanomembrane concentrators to filter exosomes from urine of healthy volunteers and proteinuric patients with focal segmental glomerulosclerosis (FSGS). The exosome isolation time was reduced from 4 h using standard ultracentrifugation to 0.5–2 h using nanomembrane filters [3]. Using this system, the authors were able to detect various proteins typically found in urinary exosomes, even from patients who had high quantities of contaminating proteins, demonstrating that this method could be used for both clinical and routine experimental applications. Other microfiltration methods have also been shown to provide an efficient way of isolating urinary exosomes with reduced contamination of highly abundant urinary proteins as compared to nanomembrane filtration and ultracentrifugation techniques [26]. Alternatively, precipitation methods have been used to isolate urinary exosomes. In 2012, Alvarez et al. [27] showed that a commercially available exosome precipitation kit called ExoQuick-TC could be used to isolate urinary exosomes, although a modified protocol provided improved results. This modified exosome precipitation method was shown to provide a more efficient yield of miRNA and mRNA than protein compared to sucrose cushion ultracentrifugation.
5.3.3 Normalization
A challenge that researchers in the urinary exosome field face is defining the methods of exosome normalization. Without standardized normalization protocols, biomarker discovery studies from urinary exosomes and the subsequent comparisons between patient-to-patient samples will be less reliable. There are several methods of normalization, including time normalization, creatinine normalization, and protein normalization.
The quantitative measurement of urinary biomarkers in terms of excretion rate is the optimal method for normalization and has been used for many years for analysis of classical urinary biomarkers such as total protein and albumin (e.g., expressed in g/day or µg/min). Assessments of urinary biomarkers based only on concentrations are unsatisfactory because normal physiological variations in water excretion can dilute or concentrate urinary proteins making this measurement unreliable for both intra- and interpersonal comparisons. Time normalization, the collection of urine from patients within the same bracket of time, is thus the most accurate method of measuring urine exosomal protein excretion rates and is the most reliable method of comparing patient exosome products side-by-side. An even more accurate time normalization would be the collection of all urine from the same patient over 24 h, but this approach has several practical limitations.
As there are difficulties acquiring time-normalized urine samples, and this method also rules out using urine samples from a biobank because these samples are typically spot urine samples, a more common method of normalization, creatinine normalization, is used in a clinical setting. Creatinine is typically excreted in urine at a steady rate. The average 24-h urine creatinine excretion rate for the general population is approximately 1,000 mg/day per 1.73 m2 body surface area. Given the amount of creatinine in the spot urine, an estimated rate of exosome excretion can be assigned. However, this method has limitations; it does not take into account actual difference in individual creatinine excretion rates or renal diseases that make creatinine excretion rates unstable, such as acute kidney disease.
Normalization based on the amount of a particular protein in urine has been proposed, including the uses of THP, exosomal markers (e.g., ALIX or TSG101), or other biomarker proteins that when measured together provide a concentration ratio that correlates with disease state [6, 28]. However, further studies are needed to confirm the validity of these normalization methods. An alternative method of normalization that has recently been utilized is counting exosome number. Using a new nanoparticle tracking analysis system, urinary exosomes in whole urine were counted, sized, and analyzed [22]. This new method of analysis may ultimately be less time-consuming and provide a more standardized normalization procedure, yet comes with the disadvantage of requiring expensive equipment.
5.4 Urinary Exosomes in Biomarker Discovery
Urinary exosomes provide a non-invasive method of discovering novel biomarkers for renal diseases. A technique that has become popular over the past decade for the discovery of novel biomarkers in the renal system and exploration of the urinary exosomal proteome is LC-MS/MS [7]. A technical description of LC-MS/MS is beyond the scope of this chapter (see review by Pisitkun et al. [29]), but a brief description is informative for the context of this section. LC-MS/MS is highly sensitive and can be used for both qualitative and quantitative analysis of peptides/proteins. Qualitative approaches involve observing the absence or presence of a particular protein in a given sample, while quantitative approaches show a comparison of the relative abundance of specific proteins. LC-MS/MS-based quantification of patient and control samples can be performed side-by-side using label-free [30], label-based (e.g., iTRAQ [31] and TMT [32]), and the more recent targeted quantification [33]. To validate and compare proteins of interest discovered by LC-MS/MS from urinary exosomes, Western blots, ELISA, or immuno-electron microscopy are commonly used.
The paradigm of utilizing a systems biology approach for clinical and translational research in the field of urinary exosome biomarker discovery is gaining momentum. The systems biology approach using mass spectrometry generally includes three phases: (1) discovery, (2) validation, and (3) implementation (Fig. 5.3) [7]. In the discovery phase, LC-MS/MS is used to identify unknown biomarkers and assist in developing hypotheses for the following phases. Typically, a limited number of well-defined patient samples are used to identify quantitative or qualitative differences in protein or mRNA/miRNA expression. Animal models are often exploited to show physiological changes within a specific experimental condition, e.g., increasing the excretion of AQP2 in urine from rats with elevated vasopressin levels by administering dDAVP or thirsting [14]. Once candidate biomarkers have been identified, the validation phase can begin.


Fig. 5.3
Systems biology workflow paradigm showing the three major steps required for the development of urinary biomarker assays for routine clinical application
A variety of promising biomarkers from urinary exosomes have been identified from many renal, urogenital, and systemic diseases. Some biomarkers of acute kidney injury (AKI), urogenital cancer, chronic kidney diseases, glomerular diseases, renal allograft rejection, and unique tubulopathies are summarized in Table 5.2. In addition to being a great source of protein biomarkers, exosomes also contain functional mRNA and miRNA, which markedly expands the potential repertoire of biomarkers. In recent studies, for example, mRNA and miRNA in urinary exosomes were used to identify potential markers for prostate cancer and renal fibrosis [34–36]. In many cases, early detection of a disease or injury is required for increasing the chances of successful treatment, and several of the biomarkers already discovered could provide early detection possibilities. For example, patients in the intensive care unit or patients undergoing heart surgery have a high risk of acute kidney injury that may increase morbidity and mortality. Proteins in urinary exosomes such as Fetuin-A [11], activating transcription factor 3 (ATF3) [10], and aquaporin-1 [37] have been shown to change significantly in the early phase of patients with AKI compared to controls and could be promising markers for early detection of AKI.
Table 5.2
Potential biomarkers for renal and systemic diseases from urinary exosomes
Groups | Diseases | Urinary exosomal proteins | Urinary exosomal RNAs | References |
---|---|---|---|---|
Acute kidney injury | Acute kidney injury (AKI)a | Fetuin-A | [11] | |
Acute kidney injury (AKI) | Activating transcription factor 3 | [10] | ||
Renal ischemia-reperfusion injury | Aquaporin-1 | [37] | ||
Cancer | Prostate cancer | PCA-3 and TMPRSS2:ERG | [36] | |
Bladder cancera | CD36, CD44, trophoblast glycoprotein, basigin, and CD73 | [40] | ||
Non-small cell lung cancer (NSCLC)a | Leucine-rich α-2-glycoprotein | [41] | ||
Renal cell carcinomaa | Matrix metalloproteinase 9, ceruloplasmin, podocalyxin, Dickkopf-related protein 4, carbonic anhydrase IX, aquaporin-1, extracellular matrix metalloproteinase inducer, neprilysin, dipeptidase 1, and syntenin-1 | [42] | ||
Bladder cancera | Tumor-associated calcium signal transducer 2 | [43] | ||
Chronic kidney disease | Renal fibrosis | microRNA-29c | [34] | |
Renal fibrosis | CD2AP | [35] | ||
Chronic kidney disease | Osteoprotegerin | [44] | ||
Glomerular disease | Puromycin-treated rats or podocin-Vpr transgenic mice and from patients with focal segmental glomerulosclerosis | Wilms tumor 1
![]() Stay updated, free articles. Join our Telegram channel![]() Full access? Get Clinical Tree![]() ![]() ![]() |