Abstract
Intestinal lipid absorption is an efficient and highly complex process. Here we review the main pathways involved in intestinal processing of dietary lipid. We discuss the regulation of enterocyte fatty acid transport and the functions in absorption of lipid transporters, scavenger receptor B1 (SR-B1), CD36 or SR-B2, and fatty acid transport protein 4 (FATP4). We describe recent findings highlighting importance of the remodeling of membrane phospholipids in particular by lysophosphatidylcholine acyltransferase 3 (LPCAT3), in facilitating passive diffusion of fatty acid and promoting triacylglycerol (TAG) assembly into lipoproteins. We review what we currently know about the most prominent intestinal enzymes involved in TAG synthesis in the endoplasmic reticulum including long-chain acyl-CoA synthetase (ACSL), monoacylglycerol acyltransferase (MGAT), and diacylglycerol acyltransferase (DGAT). We also provide a detailed and updated review on the mechanisms and regulation of prechylomicron formation, assembly, and transport from the endoplasmic reticulum to the Golgi for chylomicron generation, with special reference to the role of CD36 and liver fatty acid-binding protein (LFABP).
Keywords
CD36, SR-B1, FABP1, Prechylomicron, MGAT, DGAT
Abbreviations
ACSL
acyl-CoA synthetase long chain
ApoAI
apolipoprotein AI
ApoAIV
apolipoprotein AIV
ApoB48
apolipoprotein B48
Apobec-1
apolipoprotein B mRNA editing enzyme, catalytic polypeptide-like
COP
coatomer proteins
DAG
diacylglycerol
DGAT
DAG acyltransferase
ER
endoplasmic reticulum
FA
fatty acid
FABP
FA-binding protein
FATP
fatty acid transport protein
G3P
sn-3-glycerol phosphate
GLP-2
glucagon-like peptide-2
GPAT
G3P acyltransferase
HDL
high-density lipoprotein
Hsp
heat-shock protein
LDL
low-density lipoprotein
LPAAT
acyl-glycerol-3-phosphate O -acyltransferase
MAG
monoacylglycerol
MGAT
MAG acyltransferase
MTP
microsomal triglyceride transport protein
OEA
oleoylethanolamide
PAP
phosphatidic acid phosphatase
PC
phosphatidylcholine
PCTV
prechylomicron transport vesicle
PKC
protein kinase C
SNARE
soluble N -ethylmaleimide-sensitive factor attachment protein receptor
SR-B1
scavenger receptor B1
TAG
triacylglycerol
VAMP
vesicle-associated membrane protein
48.1
Overview of Dietary Fat Absorption
Dietary fats are the most concentrated caloric source consumed by humans. In addition to their caloric content, fats contain essential fatty acids (EFAs) and fat-soluble vitamins that are needed for health. As a consequence, humans and other animals have developed an efficient mechanism for fat absorption. In humans, 95% of a 500-g fat load can be absorbed each day, but the absorption process is quite intricate for several reasons. The majority of dietary fats are water insoluble triacylglycerols (TAGs) that must be made to interact with water in order to be absorbed by the intestine. As a corollary, lipids require specialized chaperones and enzymes with amphipathic properties in order to be processed and metabolized. In addition, potential toxicity from the fatty acids (FAs) produced during hydrolysis of dietary fat needs to be controlled. Thus, the processing of dietary fat from ingestion to output as chylomicrons is a complex story.
Dietary TAGs in the intestinal lumen are first hydrolyzed by pancreatic TAG lipase to 2 FA and one sn2-monoacylglycerol (MAG). These lipolytic products are absorbed through the apical (i.e., luminal) membrane of enterocytes, directed to the endoplasmic reticulum (ER) where they are converted back to TAG to be packaged into the intestine’s unique lipoprotein, the chylomicron. The TAG is first transported from the ER in a specialized vesicle, the prechylomicron transport vesicle (PCTV), which is directed to the Golgi where the lipoproteins of the chylomicron are processed. The mature chylomicron is then packaged into another vesicle for transport to the basolateral membrane where it is exocytosed into the intestinal lymphatic vessels called lacteals ( Fig. 48.1 A ). The chylomicron-rich lymph runs through mesenteric lymph nodes and collecting lymphatic vessels and ultimately into the thoracic duct that drains the transported lymph into the bloodstream at the left subclavian vein ( Fig. 48.1 B). The above processes will be described in detail in the following sections. This chapter is a revised updated version of the one previously coauthored with late Dr. Charles Mansbach, who made great contributions to our knowledge of chylomicron formation.

48.2
Enterocyte Uptake and Processing of FA From Luminal TAG Hydrolysis
Our understanding of the mechanisms by which FAs, generated from TAG hydrolysis in the intestinal lumen, are delivered across the enterocyte membrane and then to the ER where they are reincorporated into TAG are incomplete. However, significant progress has been accomplished and will be summarized in the following sections.
48.2.1
Enterocyte Uptake of FAs
Both diffusion and protein-facilitated membrane transfer mechanisms are likely to contribute in vivo in mediating FA uptake across the enterocyte membrane and are likely to coexist in vivo. In the circulation, FAs are carried quantitatively bound to albumin and the unbound FA concentration that is in equilibrium with albumin-bound FA (estimated to be in the low nanomolar determines the uptake by cells. In the intestine, the FA is presented to the enterocyte incorporated into bile salt micelles. Luminal micelles, like albumin in the serum, can solubilize millimolar concentrations of FA, and in both systems, the monomeric-free FA dissociated in the aqueous phase is extremely low relative to the total FA concentration. The free FA concentration in the intestinal lumen is estimated to be in the low micromolar (μM). Early studies showed that lipid constituents of micelles exhibit independent rates of cellular uptake arguing against the uptake of whole micelles. Uptake of FA from micelles appears to follow the FA monomer and in turn drives dissociation of more micellar FA.
The existence of a FA transport system at the apical surface of enterocytes was suggested by findings that metabolic processing of the FA that enters the enterocyte apically is different compared to FA that enters via the basolateral route. In addition, intestinal FA uptake exhibits variability between mice strains suggesting potential genetic determinants and shows adaptive changes in response to challenges. Over the last two decades, evidence accumulated to support protein involvement in membrane FA uptake. Saturability of the process, its sensitivity to protein reactive agents, specificity for long-chain FA and a transport Km in the low nanomolar range were demonstrated in many cell types (for detailed reviews refer to Refs. ). Most studies of FA uptake by intestinal epithelial cells used immortalized cell lines such as Caco-2 and IEC-6 as enterocyte models. Existence of saturable and nonsaturable FA uptake components was reported using either albumin:FA or micellar FA delivery systems. An interesting finding was that FA uptake could be inhibited by 2-MAG, a TAG digestion product along with FA, while triolein, glycerol, diacylglycerol (DAG), and monooctanoate had no effect. This observation suggested that enterocytes may coordinate the transport of FA and 2-MAG to optimize resynthesis of TAG inside the cell. Studies with isolated primary enterocytes from the proximal intestine of mice confirmed existence of a saturable and specific FA uptake component in these cells. However, the need to use a FA:albumin delivery assay with isolated primary enterocytes provided kinetic measures at levels of unbound FA that may be orders of magnitudes lower than those present in the intestinal lumen. Thus, the relative contribution of the protein-mediated component to total uptake by enterocytes in vivo is unclear and likely to be small as compared to cell types such as muscle or adipose cells where the saturable process may predominate. One function of the saturable FA component in enterocytes would be to serve in a regulatory capacity, transducing signals that coordinate enterocyte FA processing as discussed in following sections.
48.3
Intestinal FA Transporters
Together, the above observations supported existence of membrane proteins on the apical surface of enterocytes that might influence the uptake by recruiting the FA or contributing to regulate its intracellular trafficking to specific metabolic sites. Membrane proteins that have been proposed as potential intestinal FA transporters include the scavenger receptor CD36, the fatty acid transport protein 4 (FATP4), and the plasma membrane FA-binding protein FABPpm. Other proteins that may have relevance to FA absorption include the scavenger receptor B1 (SR-B1), which is a member of the CD36 family. FABPpm, a membrane associated form of the mitochondrial enzyme aspartate aminotransferase, was isolated from intestinal as well as liver membranes, and early studies showed that an antibody against the protein reduced FA uptake into isolated enterocytes. However, there is little additional evidence to support its role in intestinal FA uptake.
48.3.1
CD36 (SR-B2 or FAT): High-Affinity FA Uptake and Signal Transduction Regulate Absorption
CD36/FAT (FA translocase) is a 75–88-kD scavenger receptor abundant in muscle, adipose tissue, the intestine, and the capillary endothelium that recognizes a range of ligands. This includes long-chain FA, native or modified lipoproteins, thrombospondin-1, collagen, amyloid B, and malaria-infected erythrocytes. Extensive evidence, both in rodents and humans, also shows a role of CD36 in facilitating tissue FA uptake. Studies in CD36-deficient mice and humans have documented disturbances in tissue FA uptake and in overall FA metabolism. Polymorphisms in the CD36 gene have also been linked to abnormal lipid metabolism and to susceptibility to the metabolic syndrome. For reviews refer to Refs. .
48.3.1.1
Crystal Structure and Potential Mechanism for FA Transport
Significant insight into the potential mechanism for the CD36-mediated FA transport was obtained from the recently reported crystal structure of the CD36 family member lysosomal LIMP-2 and by homology that of CD36 and SR-B1. A large cavity traversing the entire length of these proteins was identified that is proposed to serve for lipid transfer to the membrane vicinity. The crystal structure of CD36 has been reported more recently, and the crystallized protein has been found to be in complex with long-chain FA with preponderance of palmitic and stearic acids. The FAs are proposed to dock within a hydrophobic pocket on the surface of the CD36 ectodomain leading toward the internal tunnel within the protein. In the surface pocket, the FA carboxylic tail is in close proximity to lysine 164 (K164), previously shown to bind the oleate derivative sulfo- N -succinimidyl oleate (SSO). Interaction of K164 with the carboxylic group of the FA could be important for its correct positioning allowing it to slide into the tunnel. Similar results were reported in the Drosophila melanogaster CD36 homologue named sensory neuron membrane protein (SNMP). Drosophila SNMP1, as well as its orthologues in other insects, is expressed in the olfactory sensory neurons that detect lipid-derived pheromones. The SNMP1 ectodomain is required for detection and binding of pheromones and the tunnel is required to funnel these molecules to their cognate receptors. K164 in the CD36 ectodomain is also involved in the binding of oxidized low-density lipoprotein (LDL). Interaction between FA and LDL for CD36 binding has been reported using surface plasmon resonance (SRP) that shows binding of the CD36 ectodomain to different long-chain FAs, including oleic acid (OA), docosahexaenoic acid (DHA), elaidic acid (EA), and palmitic acid (PA), could affect CD36 protein conformation in a manner that enhances binding of oxLDL to the receptor.
Contribution of CD36 to net intestinal FA absorption is likely to be small. CD36 can bind nanomolar concentrations of FA and would function in high-affinity uptake of FA in enterocytes of the proximal intestine, similar to its role in other cell types. At the concentrations of free FA present during absorption in the intestinal lumen CD36 will be rapidly saturated and its function would be important only in very early stages of digestion such as during the cephalic phase (see below). Consistent with this is the observation that dietary FAs downregulate levels of enterocyte CD36 protein by promoting its ubiquitination and degradation.
48.3.1.2
CD36 as a Fat Taste Receptor, Role in the Cephalic Phase of Digestion, and in Satiety
Two main classes of taste receptors for FAs have been proposed, CD36 and the G-protein-coupled receptors (GPRs). The FAs released by lingual lipase interact with the FA receptors CD36 and GPR120 expressed on the surface of taste receptor cells. CD36 is abundantly expressed on the apical surface of taste bud cells in the tongue of rodents, pigs, and humans and deletion of CD36 reduces FA preference. Consequent to long-chain FA binding, a CD36-mediated signaling cascade is triggered in taste bud cells resulting in the release of neurotransmitters and signals to the central nervous system. These events are thought to mediate fat perception, as well as the cephalic phase of digestion that primes the organism for fat absorption. With respect to the GPRs involved in fat taste perception, GPR120 is detected in gustatory and nongustatory epithelia, whereas GPR40 is not expressed in gustatory tissues, suggesting that its role in fat perception might be indirect. CD36 functions as fat taste receptor at low FA concentrations, whereas GPR120 appears to be poorly responsive to long-chain FA and might function in amplifying the response to high concentrations of dietary FA and other tastants, consistent with its expression in a variety of taste cells responsive to various stimuli.
In humans, less is known about the role of CD36 as a fat taste receptor. Several studies have investigated the role of a common CD36 polymorphism (SNP) (rs1761667 involving A/G substitution) on oral sensory fat perception. Obese African American individuals carrying the A allele of the SPN have decreased fat orosensory detection thresholds compared to noncarriers. The effect of the SNP on sensory fat perception has been replicated in other populations. A study conducted by Keller and colleagues found that subjects homozygous for the A allele, while they perceived increased creaminess in a salad dressing, were insensitive discriminators of fat content. It is possible that carriers of the CD36 SNP, which display lower FA sensitivity, reach taste “saturation” at higher FA concentrations and might be prone to increase fat intake. However, although proposed this hypothesis has not been fully tested yet.
The links between fat taste, food intake, and disease are complex, and causality has not been established. In the small intestine, CD36 is also important for fat-induced satiety via production of the messenger oleoylethanolamide (OEA), which prolongs the intermeal interval. OEA, in turn, increases intestinal CD36 expression and FA uptake. Although the above findings might suggest that CD36 absence would enhance food intake, it is actually decreased in CD36-deficient mice. This likely reflects a postingestive effect consequent to slower intestinal lipid processing as more of fat absorption is shifted to distal segments (see following sections).
48.3.1.3
CD36 Role in Fat Absorption
In small intestines of humans and rodents, the CD36 expression pattern is higher in proximal as compared to more distal segments but one study found that levels in the human ileum exceeded those in the duodenum. CD36 localizes on the apical membrane of villi (as opposed to crypt) enterocytes. The exact role of CD36 in lipid absorption is not completely clear but most evidence suggests that it functions during the early phase of the digestive process and plays a regulatory role in intestinal lipid processing. Studies in Cd36- null mice support the interpretation that the quantitative contribution of CD36 to net lipid absorption is small. Using 24-h fecal lipid recovery measurements there is no evidence of lipid malabsorption except for very long-chain FA and administration of a lipid load results in similar blood appearance of intestinally derived TAGs. These findings support the efficiency and redundancy of intestinal transport systems which easily compensate for absorption defects consequent to CD36 deficiency. CD36 expression being most abundant in the proximal intestine, a proximal defect in absorption would be expected and indeed could be demonstrated. Primary enterocytes isolated from the proximal intestine of Cd36- null mice show a 50% reduction in FA uptake as compared to enterocytes from wild type mice and in vivo intragastric administration of triolein associates with reduced OA enrichment of mucosal lipids in the proximal intestine of Cd36 -null mice. CD36 deletion also suppresses OA uptake into the duodenum and reduces it in the jejunum of mice fed after 6 h fast and then refed for 30 min. In contrast to proximal segments, a defect in FA uptake is not apparent in enterocytes isolated from the distal small intestine of Cd36 -null mice where CD36 is less abundant. Feeding of a high-fat diet results in lipid accumulation in the proximal segment with evidence for an absorption delay and for more of the lipid being absorbed distally.
During the postprandial period, CD36 undergoes rapid proteolysis involving the ubiquitin-proteasome pathway, causing disappearance of the protein from the luminal side of intestinal villi. This event is lipid-mediated and triggered by long-chain fatty acid (LCFA) and/or diglycerides derived from early digestion of dietary TAG. In a mouse model of diet-induced metabolic syndrome, abnormal chylomicron production and impaired clearance efficiency are observed. These events correlate with dysfunctional lipid trafficking and lipid sensing by intestinal CD36 most likely as a result of higher insulin. The defect in CD36 function during chronic high fat feeding in rodents was proposed to explain the secretion of a substantial fraction of smaller intestinal triglyceride-rich particle (TRL).
48.3.1.4
Role of CD36 in Chylomicron Production
There is clear evidence for a role of CD36 FA uptake and signaling in chylomicron production. Intestinal lipid secretion into the lymph measured by cannulating the mesenteric duct is 50% lower in Cd36 -null mice. The lipoproteins secreted by CD36 deficient intestines are 35% smaller and exhibit delayed blood clearance. CD36 mediates the effect of glucagon-like peptide-2 (GLP-2) to increase intestinal FA absorption and chylomicron production in hamsters and mice as the GLP-2 effect is absent in Cd36 -null mice. In addition to its facilitation of proximal FA uptake, CD36-mediated signaling contributes to other steps related to chylomicron production including enhanced expression of key chylomicron proteins such as apolipoprotein B48 (apoB48) and microsomal triglyceride transfer protein. Using an in vitro system that allows measurement of ER production of PCTVs, CD36 was identified as part of the multiprotein complex required to generate the PCTV by the enterocyte ER. In vitro PCTV budding from ER isolated from CD36 or liver fatty acid-binding protein (L-FABP) deficient enterocytes was found to be markedly defective. L-FABP binding to the ER is essential for budding of the PCTV as will be detailed in later sections.
In addition to FA, CD36 may also facilitate intestinal cholesterol absorption, which is critical for optimal chylomicron production. Enterocytes isolated from Cd36 -null mice exhibit reduced cholesterol uptake and in vivo cholesterol output into the lymph is reduced by 50%. Other findings are also consistent with CD36 functioning in intestinal cholesterol absorption. Ezetemibe treatment in mice and phytosterol (plant sterol) intake in humans, which inhibit cholesterol absorption decrease CD36 expression. Ezetimibe also inhibits CD36 facilitated cholesterol uptake in COS-7 cells. It is still unclear whether the effects of CD36 on cholesterol uptake are direct. For example, CD36 deficiency increases cellular efflux of cholesterol and phospholipids, effects which may be due at least in part to influencing membrane localization of the ATP cassette cholesterol transporter ABCA1.
CD36 is also expressed in enteroendocrine cells (ECCs), which are specialized secretory cells that release a number of peptides, including cholecystokinin (CCK) and secretin in response to FA. CCK helps optimize fat digestion by regulating gastric emptying, pancreatic secretion, intestinal motility, and gallbladder contraction. Secretin inhibits gastric emptying and synergizes with CCK to induce pancreatic secretions. The Cd36 -null mouse displays a 50% reduction in basal release of CCK and secretin and in response to gastric administration of oil. Diminished release of CCK and secretin in response to FA is also observed with CD36 deficient intestinal segments in vitro. In EEC expressing CD36 release of CCK and secretin involves FA-induced and CD36-mediated increases in calcium and the second messenger cAMP. A dysfunction in CD36 may affect secretions of these hormones resulting in a reduction of fat digestion capacity, which could contribute to more overflow of dietary lipids reaching the distal intestine.
In summary, the findings suggest that CD36 plays an important role in chylomicron production due in part to its high-affinity uptake of FA and cholesterol by proximal enterocytes. CD36-mediated signaling in response to digestion products appears to play an important role in coordinating FA and possibly cholesterol metabolic targeting for efficient lipoprotein formation. There is extensive evidence in cells other than enterocytes that documents regulated trafficking of CD36 between the plasma membrane and intracellular sites, and this may contribute to the intracellular transfer of FA and cholesterol to sites important for their packaging into lipoproteins. The possibility that CD36-mediated signaling triggered by binding FA early during digestion is important for initiating assembly of the multiprotein complex needed for formation and budding of the PCTV from the ER still needs to be tested.
48.3.1.5
CD36 Variants on Fat Absorption in Humans
Many of the findings on the role of CD36 in lipid metabolism and intestinal fat absorption in rodent models might also apply to humans. Total CD36 protein deficiency is relatively common in persons of Asian and African descent (2%–6%). Absence of myocardial FA uptake in CD36 deficient subjects has been well documented using noninvasive scintigraphy and the deficiency was shown to also associate with abnormalities of plasma lipids. Individuals with CD36 deficiency have higher levels of smaller chylomicrons, suggesting that the effect of CD36 deficiency at the level of intestinal metabolism is similar to that demonstrated in mice. Polymorphisms in the CD36 gene associate with alterations in plasma triglycerides and cholesterol in African Americans and influence susceptibility to the metabolic syndrome. In Caucasians, common polymorphisms (40%–50% incidence) are associated with high-blood FA and an increased risk of diabetes-linked cardiovascular disease.
The contribution of defective lipid processing by the small intestine to the metabolic abnormalities associated with CD36 deficiency is supported by findings of postprandial lipemia and of high levels of apoB48 in deficient Japanese subjects. In Caucasians a recent study involving close to a thousand participants found that CD36 variants that reduce CD36 expression increase levels of postprandial lipids after ingestion of a high-fat meal. Several common CD36 SNPs associated with increased levels of chylomicron remnants after the meal. In addition, associations with increased LDL particle numbers at fasting and at the post meal times were identified. Many of the associated SNPs were found to lie in close proximity to previously validated binding sites for PPARγ, a major CD36 transcriptional regulator. This gene region is characterized by active histone H3 lysine methylation and acetylation marks consistent with its importance in transcriptional regulation. In addition, DNA methylation sites that strongly reduced CD36 expression were identified and correlated with high-plasma triglycerides and LDL suggesting a potential role of epigenetic regulation of CD36 in abnormalities of lipid metabolism. The abnormal postprandial lipid profile in humans with partial or total CD36 deficiency is likely to contribute to the associations previously noted between CD36 polymorphisms and diabetes-linked cardiovascular disease and risk of metabolic syndrome.
48.3.2
CLA-1/SR-B1—Role in Intestinal Cholesterol and Possibly FA Absorption
SR-B1 is a member of the CD36 family of scavenger receptors B. SR-B1 and CD36 share several properties including binding of anionic phospholipids of native and oxidized lipoproteins and of apoptotic cells. The similarity in lipid ligands and in putative ligand-binding domains between CD36 and SR-B1 might suggest some overlap of physiological functions, but this has been examined in only a few studies. An important physiological function of SR-BI is to facilitate selective cellular uptake of cholesterol ester (CE) from high-density lipoproteins (HDLs) a task it is much more efficient at than CD36 which also binds HDL. The selective nature of CE transport suggests the existence of a nonaqueous “channel” in SR-B1 that can accommodate CE. Similar to the findings with CD36, the ectodomain of SR-B1 is important for binding the HDL-CE and probably for selective CE transfer to the internal tunnel present inside the protein. There are six cysteine residues in the C-terminal extracellular loop of SR-BI that are highly conserved and that contribute through disulfide bonds to an SR-B1 configuration that favors CE transport into the cell. However, disruption of this region does not significantly impact the ability of SR-B1 to facilitate release of free cholesterol from cells to HDL. This property is dependent on SR-B1’s N-terminal region as demonstrated using various chimeras of SR-B1 and CD36.
SR-BI tissue expression favors adrenals, ovary, and liver. However, it is also expressed in the small intestine and most studies indicate a decreasing gastrocolic expression gradient. When SR-B1 was overexpressed in the intestine in rodents, localization was found on villi and the apical surface of enterocytes of the proximal intestine. Null mice for SR-B1 did not show defects in intestinal cholesterol or FA absorption. However, transgenic mice with intestinal SR-B1 overexpression provided evidence for a role of SR-B1 in both. SR-B1 overexpression accelerated absorption of 14 C-cholesterol and 3 H-triolein. Uptake of whole triolein molecules, which was documented with SR-B1 expression in cultured cells, is unlikely to contribute significantly to these effects since dietary triglycerides are quantitatively hydrolyzed in the intestinal lumen. Based on this, it was speculated that SR-B1 might facilitate uptake of FA after hydrolysis of core triglycerides. Interestingly, SR-B1-deficient mice exhibit an increase in plasma FA similar to that observed in Cd36 -null mice and the SR-B1 effect on FA is additive to that of CD36 in mice double null for both receptors. SR-B1-mediated signaling with the activation of various kinases has been documented in response to HDL in endothelial cells and interestingly in response to postprandial micelles in recent studies. It was proposed that SR-B1 functions as a sensor of plasma membrane cholesterol and this sensing is involved in inducing apolipoprotein B cellular trafficking in response to postprandial micelles. Variants in the human CLA-1/SR-BI gene have been identified and associated with higher HDL cholesterol in healthy men.
48.3.3
FATP4: Uncertain Role in Lipid Absorption
The family of FATPs consists of six evolutionary conserved members (FATP1-6) that exhibit different tissue distribution. FATP4 is the predominant FATP present in the intestine.
The FATPs contain an ATP-binding motif that is present in acyl ligase enzymes and all have acyl-CoA ligase enzymatic activity. FATP5 has been shown to have bile acid CoA synthetase activity. The FATPs are thought of as VLCFA ligases although FATP1 and FATP4 have broad FA specificity and can activate both long and VLCFA. Expression of FATPs, especially FATP1 and FATP4, generally associates with enhanced FA uptake and FATP levels correlate with uptake under a variety of conditions. The ability of FATPs to enhance uptake is thought to reflect their acyl activity which traps FA inside the cell in a vectorial process similar to that described in bacteria. FATP4 is expressed on the intestinal brush-border as well as in the ER and subapical membranes where it traps the acyl-CoA product mediated by its ACS activity.
FATP4, the only FATP expressed in the small intestine, has been proposed to function in FA uptake by enterocytes. Such a role proved difficult to substantiate in vivo since FATP4 deletion in mice caused embryonic lethality in one study versus death soon after birth with features of restrictive dermopathy in another. A spontaneous, autosomal recessive mutation in FATP4 was associated with a restrictive dermopathy-like phenotype, confirming that FATP4 function is important for normal skin development. The role of FATP4 in intestinal absorption was examined using Fatp4 -null mice rescued for the skin phenotype and based on food consumption, weight gain, and the kinetics of intestinal cholesterol and TAG absorption, FATP4 was considered not to be important for fat absorption. Some differences were noted and these included small but significant increases in enterocyte TAG and FA content. Since FATP4 is the only FATP present in the intestine, it would be predicted that its deficiency would result in defective uptake of very long-chain FA. However, no difference in VLCFA absorption was observed although a small decrease in their levels was noted in the proximal intestine. Thus, the findings so far do not support a limiting role of FATP4 in dietary lipid absorption. Still, the protein appears linked to intestinal lipid processing as its expression is decreased by the cholesterol absorption inhibitor, ezetimibe.
48.4
Role of Phospholipid Remodeling in Intestinal FA Absorption
Recent evidence suggested that remodeling of membrane phospholipids might facilitate passive diffusion of FA into enterocytes, promoting intestinal absorption. FAs in phospholipids of cell membrane exhibit considerable diversity; saturated and monounsaturated FA are usually esterified at the sn-1 position, whereas polyunsaturated FA (PUFAs) are esterified at the sn-2 position. The asymmetrical distribution of FA at the sn-1 and sn-2 positions is maintained in part by a deacylation-reacylation process known as Lands cycle. The deacylation step catalyzed by calcium-independent phospholipase A2 removes PUFA from the sn-2 position of phosphatidylcholine (PC), which represents 70% of cellular phospholipids. The reacylation step is catalyzed by lysophosphatidylcholine acyltransferase (LPCAT), which adds PUFA at the sn-2 position of PCs. Lpcat3 -null mice die shortly after birth and when rescued by supplementing their diet with PC, the mice displayed reduced lipid absorption and lower levels of NPC1L1, CD36, and FATP4 proteins. Intestine-specific LPCAT3 deficiency led to a selective defect in incorporation of the EFAs linoleate and arachidonate into phospholipids changing the biophysical properties of the enterocyte membranes. EFA deficiency has been recognized to associate with fat malabsorption for decades, but the underlying mechanisms were never defined. Regulation of arachidonate-enrichment of membrane phospholipids by LPCAT3 is proposed to regulate passive FA transport into enterocytes. LPCAT3 would promote TAG transfer for incorporation into lipoproteins, preventing overaccumulation of cytosolic lipid droplets in enterocytes. Specifically, LPCAT3 locally enriches PUFA-containing phospholipids in the enterocyte plasma membrane. When TAG synthesis occurs in proximity, clustering of TAG is favored and a blister-like structure is formed enabling efficient TAG supply to microsomal triglyceride transport protein (MTP) at the luminal side and eventually to lipoproteins. Therefore, the lethality of Lpcat3 -null mice is attributed to the intestinal lipotoxicity caused by the intake of large amounts of milk TAG coupled with MTP inefficiency. In the setting of a TAG-rich diet, intestine-specific LPCAT3 deletion leads to cessation of food intake despite starvation probably from exacerbated production of gut hormones, including glucagon-like peptide-1 (GLP-1) and OEA. Additional studies are needed to understand whether manipulation of the intestinal membrane phospholipid composition could be useful as a strategy to modulate hyperlipidemia and diet-induced obesity. In summary, while high-affinity FA uptake and signaling mediated by CD36 serve to initiate TAG synthesis for chylomicron formation, LPCAT3-mediated remodeling of membrane lipids would promote trafficking of the synthesized TAG. Additional work is needed to determine how the two proteins might interact to coordinate chylomicron metabolism.
48.5
Liver and Intestinal Cytosolic FABP: Role in Intracellular FA Trafficking and Chylomicron Formation
Once inside the enterocyte the FA is targeted to specific intracellular sites of metabolism. The two cytosolic FA-binding proteins (FABPs) expressed in the intestine, liver-FABP (FABP1) and intestinal-FABP (FABP2) are involved in intracellular trafficking of the FA. Interestingly, although they are both from the same family and have approximately the same Mr (≈ 14 kDa), they display a number of differences. The two proteins deliver their cargo by different mechanisms. FABP1 delivers FA to membranes by diffusion and FABP2 by collision. Despite this generalization, there is some evidence that FABP1 does interact with membranes. As will be discussed in detail later, FABP1 can bind to intestinal ER to initiate transport vesicle generation during chylomicron formation. FABP2 has positive charges and an amphipathic peri-portal area suggesting a mechanism favoring membrane interaction, and a collision mechanism has been directly demonstrated using the intestine-like Caco2 cell model. Another major difference is that FABP2 binds a single FA and essentially no other lipid while FABP1 binds 2 FA rather than 1 and is more promiscuous in its ligands which include bile salts, lyso-phospholipids, cholesterol, and acyl-CoA. It is thought that FABP1 can bind 2 FA because the first FA is deep within the β barrel-binding pocket, while the second one is located more toward the surface such that its carboxyl head group can be titrated. In FABP2 and other FABPs, the one FA ligand’s carboxyl group is deep within the binding pocket. Potentially the FABPs could play a considerable role in the movement of FA from the apical membrane to the ER since they comprise 4%–6% of the cytosolic proteins in toto .
Genetic deletion of either Fabp1 or Fabp2 does not result in dietary lipid malabsorption, even with chronic high-fat feeding. In the case of Fabp1 -null mice, even on a Western diet, fat is absorbed normally although chylomicron output is modestly reduced. The reduction in chylomicron output can be explained on the basis of FABP1’s function in intracellular chylomicron trafficking as detailed later. In support of a lack of phenotype with respect to dietary fat absorption, female Fabp1 -null mice become obese. With respect to FABP2, the Fabp2 -null mice show minimal changes from wild-type (WT). Male Fabp2 -null mice gain more weight on a high-fat diet, and female mice have less weight gain than the WT mice. There is evidence that FABP1 may target FA toward oxidation while FABP2 would favor FA incorporation into TAGs. Fasted Fabp1 -null mice lose less lean mass while fasted Fabp2 -null mice lose more fat mass compared with WT mice. FABPs may exert a protective function by binding cellular FA maintaining unbound FA concentrations that are within the functional range of enzymes or receptors. Thus, a stable pool of FA and MG would contribute to optimal regulation of TAG and PL synthesis and chylomicron biogenesis. In support of this role for the FABPs, enterocyte FABP expression is also increased during high-fat feeding, presumably in response to a greater amount of FA delivered to the enterocyte.
48.6
TAG Synthesis in the ER—Important Enzymes
In the enterocyte, FA and sn-2-MAG are converted back into TAG. There are two pathways by which TAG’s immediate precursor, DAG is formed. The first one, the progressive acylation of MAG, is the primary one used for chylomicron TAG synthesis. The second or Kennedy pathway involves acylation of sn-3-glycerol phosphate (G3P) twice with FA-CoA to yield phosphatidic acid that is de-phosphorylated to DAG for use in both TAG and phospholipid synthesis. The two pathways may be geographically separated on the ER membrane, a possibility supported by data from the Tung tree.
All the acylation steps occur in the ER, which is comprised of cytosolic and luminal faces, and it is unresolved whether TAG synthesis occurs on one or both faces. If the cytosolic face is the major site, then the TAG must cross the ER membrane, a physicochemical challenge as TAG is only sparingly (3%–4%) soluble in membranes. By contrast, DAG can cross membranes rapidly and could then be used for TAG synthesis on the luminal side of the ER membrane.
When large lipid loads are infused intraduodenally into rats, nearly half the ER-associated TAG is exposed and hydrolysable by pancreatic lipase with the remainder within the ER lumen being resistant to lipolysis. The first study that addressed topology of TAG synthetic enzymes concluded they were localized to the ER cytosolic face. However, later studies suggested that the terminal enzyme in TAG synthesis, DAG acyltransferase (DGAT) is present on both sides of the ER membrane. In addition, the carnitine palmitoyl transferase-1 inhibitor, etomoxir, severely reduces lymphatic chylomicron TAG output in rats, implying that the FA-CoA may need to cross the ER membrane as an acyl-carnitine and suggesting that DAG could be acylated in the ER lumen. However, definitive evidence for this concept is still unavailable.
48.6.1
Long-Chain Acyl CoA Synthetase (ACSL)
The FA is activated by acyl-CoA synthetase long chain (ACSL) to its CoA derivative before entering TAG synthesis. There are 11 family members for common long-chain FAs and a separate enzyme, ACSVL for very long-chain FA, up to 26 carbons. In the intestine, ACLS3 and ACLS5 are the major ACLSs present ( Table 48.1 ), with a small amount of ACSL1. There is little information on specificity of the ACSL in the intestine but in the liver, ACLS3 appears specific for delivering FA-CoA for phospholipid synthesis while ACLS5 was shown to increase TAG production when expressed in McArdle-RH7777 hepatoma cells. Additionally, siRNA-mediated knockdown of ACSL5 in isolated rat hepatocytes reduced triglyceride accumulation and very low-density lipoprotein (VLDL) secretion, while increasing fat oxidation . Ablation of Acsl5 in mice antagonizes the development of obesity and insulin resistance suggesting that ACSL5 is an important regulator of whole-body energy metabolism. In addition, triglyceride absorption studies demonstrated a role for ACSL5 in dietary fat absorption.
Human | Rodents | Reference | |
---|---|---|---|
CD36 | Yes | YES | |
SR-BP1 | Yes | YES | |
FATP4 | Yes | Yes | |
FABP2 | Yes | Yes | |
MGAT1 | – | – | |
MGAT2 | – | Yes | |
MGAT3 | Ileum only | – | |
DGAT1 | Yes | Yes | |
DGAT2 | – | – | |
ACSL3 | Yes | Yes | |
ACSL5 | Yes | Yes | |
GPAT3 | Yes | Yes | |
GPAT4 | – | Yes |
48.6.2
MAG-Acyltransferase or MGAT
This enzyme acylates MAG to sn1,2 DAG and is most active in the intestine and in the neonatal rat liver. MAG acyltransferase (MGAT) is a family of three isoenzymes ( Table 48.1 ). MGAT1 is not expressed in the intestine. MGAT2 is uniquely expressed in the intestine in the mouse but also found in other tissues in humans. MGAT3 is mainly found in the ileum in humans and is not expressed in rodents. MGAT2 is expressed in a distinct proximal to distal gradient along the small intestine coincident with the site of lipid absorption. MGAT activity is important for lipid absorption since 75% of the TAG that appears in the lymph is synthesized from MAG. Consistent with this, appearance of dietary TAG in the blood after a meal is impaired in mice lacking MGAT2. Mgat2 -null mice absorb dietary fat, but the secretion of dietary TAG into the circulation occurs at a reduced rate. This appears to favor more partitioning of dietary fat toward energy dissipation, which protects the mice from high-fat-induced obesity, glucose intolerance, and fatty liver As expected, intestine-specific Mgat2 -null mice showed decreased fat absorption, similar to that observed with systemic ablation of Mgat2 genes. However, this study showed weaker antiobesity phenotypes than that of global Mgat2 -null mice, while amelioration of hepatic steatosis and insulin resistance were similar. Rescue of intestine MGAT2 expression in the Mgat2 -null mice almost completely reversed the amelioration of hepatic steatosis. A selective MGAT2 inhibitor tested in mice fed high-fat diet showed antiobesity effects through increasing energy expenditure but further studies are needed to clarify its mode of action.
48.6.3
Diacylglycerol Acyltransferase
Diacylglycerol acyltransferase (DGAT) catalyzes the terminal step in TAG synthesis by acylating DAG, no matter its origin. The intestine expresses two DGATs, DGAT1, and DGAT2. Although both enzymes have the same function, they are different in their genetic basis. DGAT1 is part of the membrane- bound O -acyltransferase (MBOT) family that includes acyl-CoA cholesterol acyltransferase (ACAT), while DGAT2 belongs to the same family as MGAT2. Additionally, DGAT1 is Mg 2 + sensitive whereas DGAT2 is not.
DGAT1, cloned in 1998 acylates in addition to DAG, retinol (acyl-CoA retinol acyl transferase [ARAT]), waxes, and importantly MAG. Dgat1 -null mice are resistant to high-fat diet induced obesity and exhibit reduced output of chylomicron-TAG. To determine if obesity resistance was a specific function of intestinal DGAT1, a mouse rescued for DGAT1 only in the intestine was generated. This mouse gained weight normally on a high-fat diet, and TAG output by the intestine returned to normal suggesting importance of DGAT1 for TAG output. Because of the favorable metabolic phenotype of Dgat1 -null mice, DGAT1 inhibitors have been developed and shown to reduce diet-induced weight gain, postprandial chylomicron secretion, and plasma total cholesterol in rodents models Similar effects have been reported in a genetic deletion of intestinal Dgat1 , which reduces postprandial triglyceride and retinyl ester excursion by inhibiting chylomicron secretion and delaying gastric empting. In a different setting, it was shown that pharmacological inhibition or ablation of Dgat1 in enterocytes affects cholesterol metabolism by reducing incorporation of CE into chylomicrons Studies in vitro using XP620 a specific inhibitor of DGAT1 suggested that 89% of the TAG formed from MAG utilized the DGAT1 pathway. In vivo XP760 reduced intestinal TAG output (measured from TAG delivery to the circulation) but only by 50%, possibly due to poor aqueous solubility of the orally administered inhibitor. When tested in clinical trials, inhibitors of DGAT2 show dose-dependent reductions in postprandial serum TAG. Significant increases in the level of plasma GLP-1 and peptide tyrosine (PYY) were seen at these doses, but no clear effect on gastric emptying was demonstrated at the end of treatment. However, the treatment was discontinued because of side effects observed in the majority of the participants. Interestingly, mutations in human DGAT1 with no detectable DGAT1 protein or activity have been described in an infant with congenital intractable diarrheal disorder, generating further concerns for the use of DGAT1 inhibitors in humans.
DGAT2 was identified in 2001 after evidence of cytoplasmic lipid droplets in cells lacking DGAT1 suggested the presence of another DGAT. Unfortunately, the Dgat2 -null mice die shortly after birth due to loss of skin barrier function and to greatly depressed plasma FA and glucose levels. The barrier function defect may be due to lack of TAG and acylceramide in the skin and the metabolic phenotype may reflect the greatly reduced adipose tissue stores. In sum, DGAT1 cannot adequately compensate for loss of DGAT2 and in the mouse most of TAG synthesis excluding the intestine utilizes the latter enzyme. DGAT2 is an integral membrane protein with two transmembrane domains and with both termini cytosolically disposed. A short (five amino acids) ER luminal component is located near the N terminus and the long C terminal domain, presumed to contain the active site, faces the cytosol. This suggests that any latent (expressed in the ER lumen) DGAT activity could be due to DGAT1. Interestingly, FA loading of cells displaces DGAT2 from the ER to lipid droplets, while DGAT1 or MGAT2 are not displaced. In addition, DGAT2 was localized to a subdomain of the ER, mitochondria-associated membranes (MAM), an area rich in lipid synthetic enzymes. In summary, DGAT1 is the primary DGAT responsible for generating TAG for chylomicrons, whereas DGAT2 plays a larger role in TAG synthesis in other tissues.
48.7
Progressive Acylation of G3P Pathway (Kennedy Pathway)
The beginning substrate, G3P, can come from a variety of sources not necessarily related to lipid metabolism and this pathway has been called the de novo pathway as well as the Kennedy pathway. It provides only a minority of chylomicron- TAG synthesis and it will be briefly discussed.
The first enzyme in this pathway, G3P acyltransferase (GPAT) acylates G3P to sn-1-lyso-phosphatidic acid and includes four family members of which two, GPAT3 and GPAT4 are present in the ER ( Table 48.1 ). In the intestine, GPAT3 has the highest expression other than white adipose tissue while GPAT4 is expressed in the intestine at a more modest level compared to other tissues. Whether any of them play an indispensable role I intestinal TAG synthesis is yet to be explored.
The second enzyme is acyl-glycerol-3-phosphate O -acyltransferase (LPAAT), which acylates lyso-phosphatidic acid to phosphatidic acid and includes two family members and an LPAAT3 was recently added. LPAAT1 and 2 are present in the intestine. This enzyme not only provides phosphatidic acid for the next step on the synthetic pathway but is also crucial in the Lands phospholipid remodeling cycle. In this cycle, phospholipase A2 hydrolyzes the sn-2 acyl group of phosphatidic acid to sn-2-lyso-phosphatidic acid, which is then replaced with a different FA at the CoA level by LPAAT. In this way, membranes are able to keep the FA constituents of the phospholipids at their required melting points. Recently, in liver, LPAAT1 has been shown to have MGAT activity providing another potential pathway for DAG formation. The conversion of phosphatidic acid to DAG is a function of phosphatidic acid phosphatase (PAP). The finding that PAP’s activity affects a number of lipid synthetic enzymes at the nuclear level have resulted in a change in the name of PAP to lipin. There are 3 lipin family members with lipin2 and 3 expressed in the intestine. The activity of lipin is controlled by phosphorylation. Unlike other lipid synthetic enzymes, lipin is a cytosolic enzyme where it is inactive when phosphorylated. On dephosphorylation, it is displaced from the cytosol to the ER membrane where it becomes active.
48.8
Distinct Intracellular TAG Pools in the Enterocyte
There are at least two pools of intracellular TAG in enterocytes ( Fig. 48.2 ). The first is thought to be in the ER lumen and a precursor of chylomicron-TAG. The second pool is suggested to be in intracellular lipid droplets that exit the intestine either as TAG or FA via the portal vein. MAG and FA entering the enterocyte via the apical membrane are preferentially shunted into the chylomicron-TAG precursor pool. When glyceryl-tri- 3 H-oleate was infused intraduodenally into rats, the 3 H-TAG in the mucosa had a specific activity of only ≈ 30% of that of the infusate indicating significant dilution with endogenous TAG. By contrast, the specific activity of chylomicron-TAG approached that of the infusate. By contrast, if the 3 H-oleate was given intravenously, the oleate entered an intracellular TAG pool that contributed minimally to chylomicron-TAG. Further, the distribution of FA or MAG entering by the apical versus the basolateral route is changed from that favoring a TAG end product to one contributing more to phospholipid synthesis or being oxidized. Consistent with separation of the TAG pools is that once the TAG has entered the ER lumen, if its exit is blocked by the nonionic detergent pluronic L-81 (L-81) upon relief of the blockade it appears in the lymph as chylomicron-TAG.

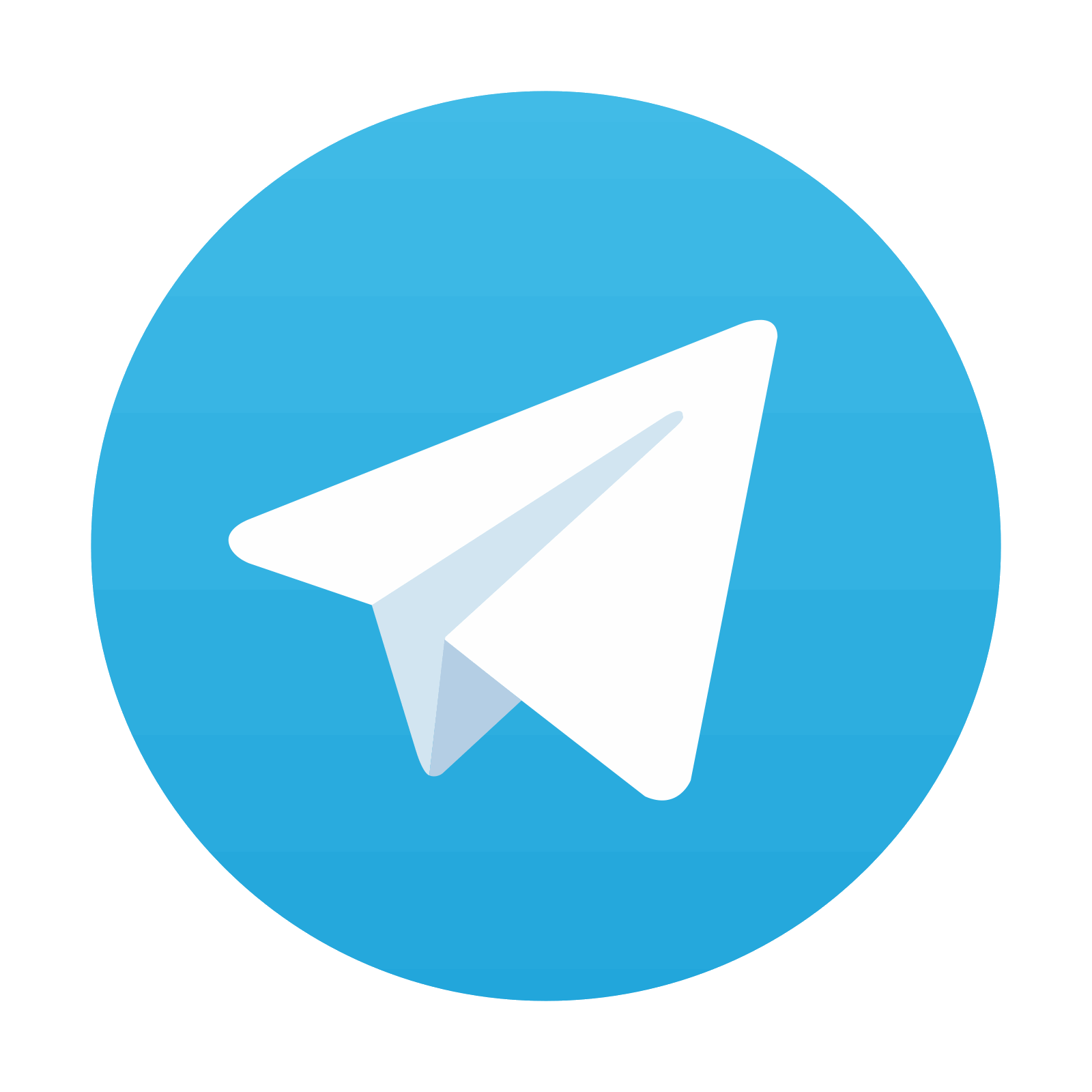
Stay updated, free articles. Join our Telegram channel
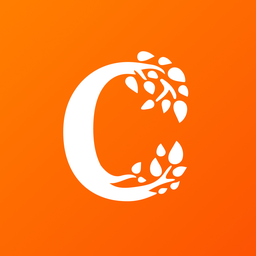
Full access? Get Clinical Tree
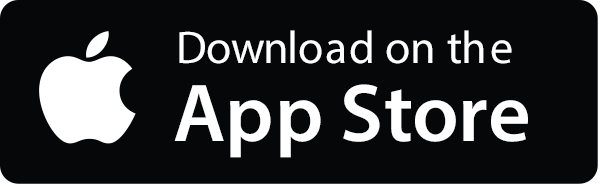
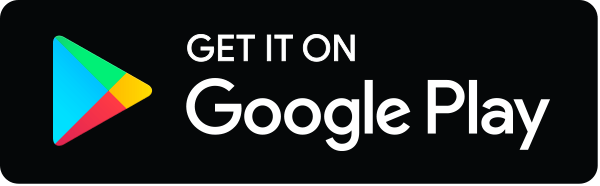
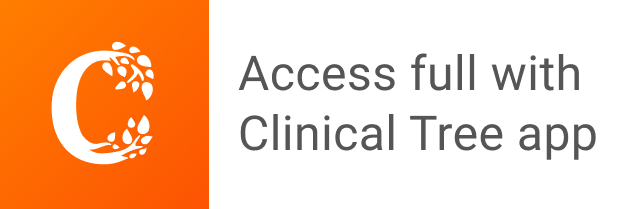