Abstract
Optical coherence tomography (OCT) is an optical analog of ultrasound imaging that was invented in the early 1990s. OCT fills a resolution gap between confocal laser endomicroscopy (CLE) and ultrasound, affording the capability of visualizing architectural microscopic morphology at a resolution of approximately 10 µm and to a depth of several millimeters (mm). OCT is also advantageous in that it provides cross-sectional images that are similar to that of histopathology viewed under low power magnification. Similar to ultrasound, OCT measures the time delay of optical echoes backscattered from structures within tissue, capturing microstructural data as a function of depth within tissue.
Keywords
Optical coherence tomography, confocal laser endomicroscopy, GI tract, Barrett’s esophagus, tethered capsule endomicroscopy, computer-aided diagnosis
8.1
Introduction
Optical coherence tomography (OCT) is an optical analog of ultrasound imaging that was invented in the early 1990s . OCT fills a resolution gap between confocal laser endomicroscopy (CLE) and ultrasound, affording the capability of visualizing architectural microscopic morphology at a resolution of approximately 10 µm and to a depth of several millimeters (mm). OCT is also advantageous in that it provides cross-sectional images that are similar to that of histopathology viewed under low power magnification. Similar to ultrasound, OCT measures the time delay of optical echoes backscattered from structures within tissue, capturing microstructural data as a function of depth within tissue.
8.2
Principles of Optical Coherence Tomography
OCT uses near-infrared (NIR) light that cannot be seen by eye. The time delay of NIR light backscattered from tissue is measured using an optical method called interferometry ( Fig. 8.1 ). After emanating from the light source, it is split so that some of it travels to the patient in the so-called sample arm through an imaging probe. The remaining light travels roughly the same path length in a reference arm where it is redirected back toward the system. Sample arm light returned from the tissue is combined with the reference arm light and detected by one or more detectors. If the distances that the light in the sample and reference arms have traveled are approximately equivalent, high and low intensities are observed by the detector, a pattern known as interference. The interference pattern is analyzed by electronics inside the OCT console to measure tissue reflectance as a function of delay time or depth that the sample arm light has propagated within the tissue. This depth-dependent reflectance profile is commonly known as an A-line or A-scan. Cross-sectional images are acquired by recording A-lines as the sample arm beam is scanned across the sample by moving the optics in the probe.

There are multiple types of OCT systems that have been used for imaging the esophagus, the first-generation time-domain OCT (TD-OCT) and more recent second-generation Fourier-domain OCT (FD-OCT) systems, also known as spectral-domain OCT (SD-OCT) , or swept-source OCT (SS-OCT) , which has also been termed optical frequency-domain imaging (OFDI) . The primary functional difference between TD-OCT and FD-OCT systems is that FD-OCT systems obtain images at a much faster rate than TD-OCT systems. This speed advantage enables three-dimensional microscopic imaging of large regions of the esophagus, a capability that has been recently termed volumetric laser endomicroscopy (VLE) .
8.2.1
Optical Coherence Tomography Parameters
OCT produces cross-sectional images in depth and along a lateral dimension. As a result, there are two axes of resolution that govern the quality of the OCT image. The first, termed axial resolution, is measured along the depth axis and is proportional to the spectral bandwidth of the source. For typical OCT systems with bandwidths around 100–140 nm, the axial resolution is approximately 5–10 µm in tissue. Along the scanning trajectory that is generally perpendicular to the axis of the light beam, the lateral or transverse resolution is defined by the focusing power of the lens in the sample arm probe. Typical values for the lateral resolution are 30–50 µm.
Ranging depth is defined as the axial (depth) distance over which the image is acquired. Typical ranging depths for today’s OCT systems are around 6 mm in air or 4.5 mm in tissue. Penetration depth is defined as the distance inside tissue over which an OCT signal can be obtained. This distance depends on the amount of attenuation that the light undergoes as it propagates through the esophageal wall. Generally, the penetration depth in esophageal tissues ranges from 1 to 3 mm, but the penetration depth may be smaller for example in invasive cancer, gastric cardia, or esophageal cardiac metaplasia.
Contrast in OCT comes from backscattering of different cellular and extracellular tissue constituents. The strength of the backscattering and therefore the OCT signal is based on a property of the cellular components called refractive index that governs the speed of light propagation in a medium. When refractive index differences are encountered in tissue, this interface scatters light; the greater the refractive index difference, the greater the scattering. Another factor that governs backscattering strength and thus the OCT signal is the size and concentration of scatterers. Larger scatters and higher concentrations of scatterers provide a higher OCT signal than smaller and lower concentrations of scatterers. For instance, since nuclei are large relative to other organelles and have a relatively high refractive index gradient , large nuclei such as those encountered in high-grade dysplasia (HGD), typically can give rise to a high OCT signal.
The speed of the OCT system is defined by the A-line rate, or the time required to acquire a single depth resolve backscattering profile. Depending on the A-line rate, the scanning mechanism, the scanning pattern, and the required sampling density, various two-dimensional frame rates and three-dimensional volume rates can be achieved. Many recent endoscopic OCT systems perform high-density radial scanning that provide circumferential cross-sectional images of the entire esophageal wall that are displayed in real time. These systems sample one or two times per lateral resolution element along the circumference, so for example for a 20 mm diameter balloon-centering catheter in esophagus, the number of A-lines required for a single cross-sectional image may be between 2000 and 4000. For VLE, a helically scanning method is used where the cross-sectional images are acquired as the rotating optics in the probe are pulled back. Typical VLE scans of the esophagus can span 6 cm, acquiring three-dimensional OCT datasets in 30–60 seconds . More rapid scans used for positioning the probe in VLE, so called scout scans reduce this time significantly by increasing the pullback rate.
8.3
History of Optical Coherence Tomography in the Esophagus
8.3.1
First-Generation Esophageal Time-Domain Optical Coherence Tomography
The first OCT images of gastrointestinal (GI) tract tissues ex vivo were obtained in late 1990s, demonstrating the potential of this technology to visualize the microscopic architectural morphology of GI tract mucosa . First internal organ imaging in vivo followed the development of a completely new concept for a fiber-based imaging probe with miniaturized focusing optics . The first endoscopic OCT catheter was developed and tested in in vivo experiments in rabbits at the Massachusetts Institute of Technology (MIT) . The catheter comprised a 2 mm transparent sheath capable of being inserted into a working channel of an endoscope. The sheath enclosed an optical probe that contained an optical fiber surrounded by a cable or driveshaft. The optical fiber was terminated at the distal tip by a lens and a prism for focusing and redirecting the light perpendicular to the axis of the probe . Images could be obtained by rotating the driveshaft while recording sequential OCT A-lines. Images of the rabbit esophagus and trachea in this study showed remarkable detail in vivo, paving the way for future developments and human application of endoscopic OCT. The first human results with endoscopic OCT were obtained by Sergeev et al. using another type of the probe that imaged in the forward direction over a field of approximately 2 mm. In this study, images were obtained in normal subjects and patients with esophageal disorders. Side-scanning probes with larger fields of view, developed by other laboratories, were subsequently demonstrated in human esophagus in vivo and reported a few years later .
Following these first in vivo human studies using endoscopic OCT, the technology entered a second phase of development focused on interpretation of OCT images obtained in human tissues both ex vivo and in vivo in comparison to histopathological diagnosis . Performance of endoscopic OCT was also compared to a high-frequency endoscopic ultrasound (EUS) probe showing that the resolution of OCT was much higher than that of EUS .
In early 2000s, the first OCT image feature criteria for Barrett’s esophagus (BE) and dysplasia were published based on co-registered endoscopic OCT images and biopsy samples obtained from patients at different stages of the disease. Criteria were developed using training sets and the sensitivity and specificity of the developed criteria were tested prospectively on new images using the histopathological diagnoses as the gold standard. Using criteria (see the paragraph entitled: “Optical coherence tomography images of the esophagus” for a description of the criteria) derived from TD-OCT images, sensitivities from 81% to 97% and specificities from 57% to 92% were determined for diagnosing BE (defined as specialized intestinal metaplasia (SIM)). Sensitivities from 54% to 83% and specificities from 72% to 75% were obtained for detection of high-grade dysplasia and intramucosal carcinoma. The large spread of results can in part be explained by the use of first-generation TD-OCT technology and research-grade prototypes, which did not provide images that have the high quality seen from second-generation systems, and by incorrect co-registration of biopsy samples caused by the need to sequentially image, remove the imaging probe, reinsert the biopsy forceps, and then biopsy the same location in the esophagus.
Along with the progress on understanding the diagnostic performance and the clinical potential of OCT for the diagnosis of BE, many research groups were focused on technical development of OCT systems to further improve quality of images. At that time, the majority of studies with TD-OCT were performed with semiconductor light sources providing axial resolution of 10–15 µm. Larger bandwidth sources based on femtosecond lasers were developed, making it possible to image with a much higher axial resolution . In 2007, Chen et al. published a study comparing standard resolution endoscopic OCT with an ultrahigh-resolution OCT system that used a femtosecond laser. While sensitivity and specificity were not reported, this group showed that the higher resolution provided better quality images and improved the visualization of finer architectural details. Because of the size and cost of these more complex lasers, most clinical endoscopic OCT systems still use semiconductor light sources.
8.3.2
Second-Generation Esophageal Fourier-Domain Optical Coherence Tomography
In comparison to the standard of care in the GI tract, OCT provides optical biopsy without need of tissue resection permitting better sampling of the organ. However, data acquisition speed of the TD-OCT systems was not sufficient for inspection of larger areas; therefore, this first-generation OCT technology was primarily limited to point sampling. Introduction of FD-OCT significantly increased OCT imaging speed and opened up new possibilities for imaging the entire distal esophagus. In order to image the entire esophageal circumference, the optical probe had to be positioned near the center of the lumen in order to keep the tissue in focus. In 2006, the OCT group at Massachusetts General Hospital developed a balloon-centering probe to assure correct location of the tissue with respect to the probe and, using a driveshaft for rotation and translation of the optics, conducted helical scanning to capture three-dimensional FD-OCT images over a 6 cm length of esophagus. New OFDI/SS-OCT technology, including a high-speed wavelength swept laser , was developed to enable high A-line rates (up to 54 kHz) and decrease acquisition time further . This technique was called comprehensive volumetric microscopy , which subsequently has become synonymous with VLE . Balloon-catheter OFDI was then conducted in patients undergoing endoscopy, demonstrating that this technology was capable of providing high-quality, three-dimensional OCT images of the entire distal esophagus in living patients . A portion of this dataset was used by Sauk et al. to test intraobserver agreement for the diagnosis of BE with VLE. Ten readers were first trained using BE diagnostic criteria previously developed in TD-OCT studies and then asked to score another set of images. An excellent agreement for differentiation of intestinal metaplasia versus non-BE was achieved with a κ value of 0.811 (95% confidence interval (CI) 0.73–0.89; p <0.0001) . Subsequently, in 2011, NinePoint Medical commercialized an OCT VLE system and a balloon-centering catheter that can be inserted into the accessory port of the endoscope. Following FDA approval in 2012, the device has been utilized in hundreds of patients to date. Most recently, a report by Wolfsen et al. from multicenter experience with 100 patients has been published showing that VLE is safe and feasible for introduction into a clinical practice.
During the development of OCT VLE, a question was raised as to whether or not the pressure of the balloon on the esophageal wall influenced the appearance of tissue architecture seen by OCT. This question was investigated by Kang et al. in 2010, who developed a double balloon catheter, where images were obtained either in between two balloons or through one of the balloons. In a preliminary in vivo study in swine esophagus, it was found that topographic information from mucosal surface was sometimes lost due to balloon compression. It was also noted that the presence of the balloon decreased visibility of certain internal structures like vessels but increased imaging penetration depth so that the muscularis propria could be more easily seen .
In parallel to the development of the balloon-centering catheters, other groups continued to develop and utilize small diameter, radially scanning probes that are inserted in the endoscope accessory port and placed in close contact with the esophageal wall prior to imaging. These probes provide partial coverage of the esophageal circumference in a single longitudinal pullback; greater coverage can be achieved by repositioning the probe using the endoscope. While a smaller tissue region is imaged using these devices, the much shorter working distance of these probes facilitates image generation with higher transverse resolution providing very high-quality images of various esophageal structures and diseases .
8.4
Optical Coherence Tomography Images of the Esophagus
8.4.1
Normal Esophageal Squamous Mucosa
Five anatomic layers of the normal esophagus can be easily distinguished in OCT images ( Fig. 8.2a,e ): the epithelium, lamina propria, muscularis mucosa, submucosa, and muscularis propria. The intensity of the OCT signal alternates between the layers due to difference in their optical properties, which allows for clear delineation of the stratified morphology. Of note, the muscularis mucosa are distinct in images of squamous mucosa obtained with endoscopic probes used in TD-OCT ( Fig. 8.2a ) whereas oftentimes, it is merged with the lamina propria in images acquired with balloon-centering VLE catheters. Additional structures like submucosal glands, mucin ducts, or vessels can be also found in OCT images.

8.4.2
Stomach
When VLE technology was introduced, it enabled volumetric imaging of the whole distal esophagus including the folds of the gastric cardia. It is a common approach for diagnosing BE in the volumetric OCT dataset to first localize cardia as a distal landmark and then inspect consecutive, more proximal images for presence of specialized intestinal metaplasia. Normal gastric tissue has a very typical appearance in OCT images ( Fig. 8.2b,f ) with the following features: (1) fine, superficial vertical structures of alternating low and high OCT signal corresponding to gastric pits, (2) much lower penetration depth, and (3) gastric rugae.
8.4.3
Barrett’s Esophagus
Even though conventional OCT does not provide high enough resolution to resolve goblet cells, studies have shown that this technology is capable of differentiating SIM from other upper GI tissue types. In a study by Poneros et al. , 166 OCT images were compared to histology of co-registered biopsy specimens. Using this data, it was determined that at least two of the following characteristic features had to be present for differentiating SIM ( Fig. 8.2c,g ) from normal squamous epithelium and normal gastric mucosa: (1) lack of layered morphology of normal squamous tissue and absence of “pit and crypt” structures of normal gastric mucosa, (2) heterogeneous, disorganized intensity of the OCT signal within the tissue and irregular surface topography, and (3) presence of glands below the epithelial surface. The lack of normal squamous or gastric morphology and heterogeneous tissue backscattering were published as the most important criteria. In another study published by Sauk et al. , heterogeneity and irregular surface topography most frequently lead 10 readers (5 gastroenterologist, 1 pathologist, 4 OCT experts) to render a diagnosis of BE.
8.4.4
Dysplasia in Barrett’s Esophagus
The most comprehensive study on identifying dysplasia in BE ( Fig. 8.2d,h ) has been published by Evans et al. . A scoring system for dysplasia progression was developed and tested in 177 biopsy-correlated TD-OCT images. For each OCT image, a dysplasia index was calculated based on a sum of scores for the following two features: (1) surface maturation and (2) gland architecture. Poor surface maturation is a histological feature related to an increase in the nuclear to cytoplasmic ratio of the surface epithelium. Because refractive indices of the cytoplasm and chromatin are significantly different, an increase in nuclear size and density increases the intensity of the OCT signal at the surface of the tissue. If the OCT signal from the surface was weaker than the signal from the subsurface, it had a score 0; if equivalent, the score was 1; and if the surface OCT signal was stronger, then the score was 2. A similar scoring was defined for appearance of glands in the OCT image: a normal glandular architecture with typical linear structures with alternating intensity of OCT signal and minimal number of smooth dilated glands/ducts was given a score of 0; more irregular glandular architecture and an increased number of dilated glands/ducts had a score of 1; and high glandular irregularity with glands that were back-to-back or had highly asymmetric shapes or contained debris were given a score of 2. A summed dysplasia index that was equal or larger than 2 was found to be 83.3% sensitive (95% CI 70–93) and 75.0% specific (95% CI 68–84) for the diagnosis of high-grade dysplasia or intramucosal carcinoma.
Since FD-OCT and TD-OCT images are similar in appearance, it is probable that a form of these TD-OCT criteria will apply to VLE data but this remains to be demonstrated in the literature in vivo, as validation of VLE has only been conducted ex vivo to date. Leggett et al. investigated capabilities of VLE for detection of dysplasia associated with BE. In this study, endoscopic mucosal resection (EMR) specimens from 27 patients with BE were used to determine the sensitivity and specificity of VLE compared to histology. The findings of this study showed a sensitivity of 86% (95% CI 69–96), specificity of 88% (95% CI 60–99) and an overall diagnostic accuracy of 87% (95% CI 86–88) . In another study, difficulties with one-to-one correlation between VLE and histology using EMR specimens was addressed by Swager et al. where various combinations of marker placement and tissue block sectioning were investigated for improved correlation.
8.4.5
Response to Esophageal Ablation
Following esophageal ablation, squamous epithelium can be found overlying Barrett’s epithelium , which may be of concern if this residual Barrett’s has neoplastic potential. OCT has considerable advantages over video endoscopy with respect to the assessment of subsquamous BE as it provides microscopic information deep into the esophageal wall ( Fig. 8.3 ). OCT images of buried Barrett’s were first reported in vivo in a case study by Adler et al. . Application of OCT for detection of subsquamous BE was further investigated by Cobb et al. where esophagectomy specimens were collected from 14 patients and immediately imaged with an ultrahigh-resolution benchtop OCT system before histological processing. Co-registered OCT and histology data showed subsquamous BE that appeared by OCT as glands underneath neosquamous epithelium. In this study, it was noted that it could be difficult to differentiate Barrett’s glands that appeared to have a thick double-walled appearance from vessels with thinner walls . Later, Zhou et al. performed a study where OCT volumetric data was obtained from 18 patients before complete eradication of intestinal metaplasia and 16 patients post-eradication. In their analysis of OCT cross sections, buried glands with irregular size and shape and sparse distribution were differentiated from tube-like vessels and from well-organized esophageal glands below the lamina propria/muscularis mucosa. A total number of 620 buried glands were found in 72% of patients (13/18) before complete eradication of intestinal metaplasia, this number decreased to 114 buried glands found in 63% of patients (10/16) after the procedure; however, no significant change in size or distribution was found. However, due to the lack of histological correlation, it was impossible to definitively confirm that the structures identified were buried BE glands. In the most recent study with a VLE system published by Swager et al. , many post-ablation buried Barrett’s regions identified with the VLE corresponded to normal histological structures like dilated glands and blood vessels. In this study, the VLE system was first used to identify areas of buried Barrett’s during EGD procedure (found in 13 out of 17 patients), and in a second step, the tissue was imaged ex vivo after the area was excised. Only one area identified by VLE as positive was also found to contain buried Barrett’s on corresponding histology ( Fig. 8.3 ). While these studies have confirmed that OCT can be used to visualize subsquamous microscopic structures, further research is necessary to develop criteria specific to subsquamous Barrett’s that can be useful for surveillance of post-ablation patients.
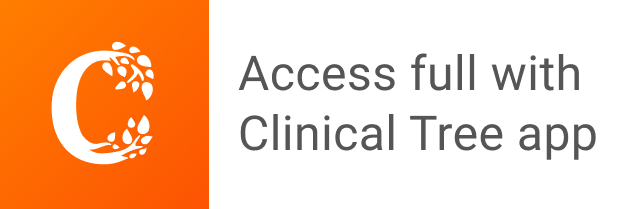