Fig. 5.1
Protein synthesis system: Protein production is regulated and orchestrated by two signaling pathways: mTORC1 and UPR. Both pathways require ATP as an energetic currency, in which mTORC1 activation is the most energy-consuming pathway
5.2 Factors of Protein Synthesis Mechanisms in Podocytes
5.2.1 Adenosine Triphosphate (ATP) as Energy Currency in Podocytes
All living cells use ATP as a biological energy currency to perform the large number of intra- and extracellular events involved in metabolism, cell cycle, membrane transport, mechanical movement, and secretion. The main fuel of ATP production is glucose, which is processed by glycolysis in the cytoplasm and by the Krebs cycle and oxidative phosphorylation in the mitochondria. The respiratory chain complexes utilize NADH to generate a proton gradient in the inner mitochondrial membrane, enabling the production of large amounts of ATP by mitochondrial ATP synthase [3]. A measurement of oxygen consumption rates and extracellular acidification rates in a transformed mouse podocyte cell line using the Seahorse Bioscience XF24 Extracellular Flux Analyzer indicated that mitochondria play the primary role in maintaining podocyte energy homeostasis, while glycolysis provides a lesser contribution [4]. Accumulated evidence has revealed the clear cross talk between podocyte mitochondrial dysfunction and glomerular proteinuria. For instance, an A to G transition at position 3243 in mitochondrial DNA (A3243G) is associated with myopathy, encephalopathy, lactic acidosis, and stroke-like episodes (MELAS) and progressive external ophthalmoplegia (PEO) [5]. However, many patients with the A3243G mutation initially present with nephrotic syndrome, without any sign of MELAS and PEO [6–8]. The pathogenesis of glomerular proteinuria in such patients could be explained by podocyte injury. Their glomerular pathology indicates focal segmental glomerulosclerosis, a typical podocyte disease. These reports suggest that the podocyte energy system plays an indispensable role in the integrity of the glomerular permselective barrier.
5.2.2 Glucose
Although glucose is a major fuel for intracellular ATP as described above, it is unclear whether hypoglycemia itself leads to podocyte dysfunction and causes glomerular proteinuria. To explore this question, it is essential to develop an animal model exhibiting long-term hypoglycemia. On the other hand, more than three decades ago, one preliminary clinical trial studied in juvenile diabetics. In that study, the patients were treated with intravenous insulin in combination with glucose infusion to maintain blood glucose levels. The results were intriguing in that urinary albumin excretion approximately doubled after insulin infusion, from 6.8 to 12.5 microgram/min, without a significant change in the glomerular filtration rate or renal plasma flow [9]. Recently, mice with a specific deletion of the insulin receptor from podocytes developed significant albuminuria together with histological features that recapitulated diabetic nephropathy, but in a normoglycemic environment [10]. The authors further demonstrated that an insulin signaling cascade in podocytes through the mitogen-activated protein kinase (MAPK) and phosphoinositide 3-kinase (PI3K) pathways via the insulin receptor directly remodels the actin cytoskeleton [10]. Although the data between these two studies may appear contradictory, it is clear that pathways downstream of insulin signaling exist in podocytes. These may underlie the physiology and pathology of glomerular proteinuria.
Since the 1990s, molecular and functional cloning methods have identified many transporter families for each nutrient (refer to SLC TABLE: http://www.bioparadigms.org/slc/intro.htm). Glucose is taken into cells through the glucose transporters (GLUTs) at the plasma membrane, of which there are currently 14 known members [11]. Although studies revealed GLUT1, GLUT3, GLUT4, and GLUT8 to be expressed in the glomerulus, whether these isoforms indeed exist and function in podocytes and how they are involved in energy production under physiological and pathological conditions require additional studies [12]. GLUT4 is the most studied isoform in podocyte biology. Glucose uptake by insulin in podocytes is conducted through the translocation of GLUT4 and GLUT1, depending on the filamentous actin cytoskeleton [13]. Podocyte-specific GLUT4-deficient mice do not develop proteinuria, despite having larger and fewer podocytes than wild type, and are also protected from diabetes-induced hypertrophy due to the failure to activate mTOR [14]. In contrast, GLUT4-deficient mice treated with the mTOR inhibitor (mTORI) rapamycin developed worse adriamycin-induced nephropathy than WT mice [14]. This contradiction regarding the altered podocyte sensitivity to different insults in GLUT4-deficient mice indicates the complicated interaction between glucose and the signaling pathway.
5.2.3 Amino Acids
Amino acid intake across the plasma membrane is mediated by amino acid transporters. The L amino acid transporter (LAT) family prefers branched-chain amino acids (BCAA) as their substrates [15, 16]. Four LATs (LAT1–LAT4) have been identified, and LAT3 is the only amino acid transporter that locates at the plasma membrane and responds to amino acids in podocytes. LAT3 was first identified by expression cloning in a human hepatocarcinoma cell line [17]. We thereafter proved that LAT3 is sensitive to nutritional depletion in the liver and skeletal muscle [18]. In addition, it was clearly revealed that LAT3 is one of the major routes to provide podocytes with BCAAs. The effect of nutrition on podocyte LAT3 is intriguing. Food starvation upregulates LAT3 and phosphorylated oncogene identified in the AKT8 retrovirus (AKT) 1 (AKT1, S473) in podocytes, accompanied by the reconstitution of the actin network and foot process elongation [19]. However, the direct relevance of LAT3 function in podocyte energy metabolism remains to be further elucidated. Despite LAT1’s known expression in malignant cells [20–22], its plasma membrane expression in normal tissues has only been reported in the vascular endothelial cells forming the blood–brain barrier [23, 24] and syncytiotrophoblastic cells of the placenta [25]. LAT2 is expressed in the plasma membrane of the normal epithelium of the kidney proximal tubules and the digestive tract [26–30], while LAT4 locates to the renal distal tubules and collecting ducts [31]. Importantly, both LAT1 and LAT2 require binding to 4 F2 cell-surface antigen heavy chain (4F2hc) to form a heterodimeric complex via disulfide bonds, which is essential for their localization in the plasma membrane [30, 32]. We revealed that both LAT1 and LAT2 exist in the cytoplasm but not in the plasma membrane in normal podocytes [33]. The absence of plasma membrane localization of both isoforms is possibly explained by the lack of 4F2hc protein in normal podocytes. Interestingly, in the model of crescentic glomerulonephritis, both LAT2 and 4F2hc mRNA are upregulated in podocytes as well as in Bowman’s cells, and their proteins are relocated to the plasma membrane. This action occurs prior to the crescent formation [33]. This phenomenon suggests that LAT2 plays a biological role underlying cell proliferation in glomerulonephritis, but it is still unclear whether this isoform also underlies the pathogenesis of proteinuria in nephrotic syndrome.
5.2.4 Nucleosides
Nucleosides are salvaged back to nucleoside triphosphates including ATP, guanosine triphosphate (GTP), cytidine triphosphate (CTP), 5-methyluridine triphosphate (m5UTP), and uridine triphosphate (UTP) in the cell cytosol, with the exception of inosine, whose salvage is limited to inosine monophosphate. Nucleoside triphosphates supply energy and phosphate groups for phosphorylation and therefore play a fundamental role in the physiology of many organs. Nucleosides also require their own transporters for their import into the cell cytosol. Mammalian cells possess two major nucleoside transporter families: the equilibrative nucleoside transporters (ENTs) mediate nucleoside transport in both directions depending on the nucleoside concentration gradient across the plasma membrane, and the concentrative nucleoside transporters (CNTs) mediate nucleoside transport independent of the nucleoside concentration gradient across the plasma membrane [34–37]. Although the mRNA expression pattern of the nucleoside transporters varies among organs and species [38], protein expression in organs including the kidney remains largely unsolved. It is reported that the mRNAs of CNT isoforms 1–3 are expressed through the rat nephron, and CNT 2 is the predominant isoform in the glomerulus [39]. We further identified the protein of this isoform expressed in the podocytes of the human kidney glomerulus [40]. Adenosine is the main nucleoside relevant to energy metabolism, which is taken up by mammalian cells through ENT1 and CNT2. Adenosine activates adenosine monophosphate-activated kinase (AMPK), a central sensor of the intracellular energy status [41–43]. Thus, CNT2 in podocytes seems to play a fundamental role in glomerular physiology and pathology by regulating the intracellular adenosine content through controlling AMPK activation.
Both quiescent and damaged cells release ATP outside the cell. Those cells recognize extracellular ATP through P2X ATP receptors, which in turn induce autocrine purinergic signaling activation [44]. Ectonucleotidases rapidly hydrolyze ATP to ADP, AMP, and adenosine on the cell surface [45], which in turn activate many signaling pathways through binding to P2Y nucleotide receptors and P1 adenosine receptors [44]. The reuptake of adenosine via nucleoside transporters (ENT1 and CNT2) is important for ATP generation inside cells [41]. Podocytes express P1 adenosine receptors (A1 and A2a) in the rat kidney [46, 47]. However, how these receptors are involved in the pathogenesis of glomerular proteinuria in nephrotic syndrome remains unknown.
5.3 Regulatory Mechanism of Protein Synthesis
5.3.1 Energy-Consuming Process: Mechanistic/Mammalian Target of Rapamycin (mTOR)
The maintenance of protein expression in a cell is tightly regulated by an equilibrium between synthesis and degradation. Protein synthesis occurs via the specific signaling pathway activated through mTOR (mechanistic target of rapamycin, formerly known as the mammalian target of rapamycin). mTOR is an evolutionarily conserved serine/threonine kinase that was first identified from dominant missense mutations in yeast resistant to the immunosuppressant rapamycin [48, 49]. mTOR forms two functional complexes, mTOR complex1 (mTORC1) and mTOR complex2 (mTORC2) [50]. mTORC1 contains mTOR, raptor, mLST8, and PRAS40, whereas mTORC2 is composed of mTOR, rictor, mLST8, mSin1, and protor [51–54]. The activation of the mTORC1 pathway stimulates both the initiation and elongation phases of mRNA translation and increases ribosome biogenesis, thereby accelerating cell size enlargement and cell proliferation [55]. mTORC1 activation occurs through sensing upstream stimuli, including nutrients (e.g., amino acids), growth factors (insulin), and the cellular energy status (ATP/AMP ratio) [56]. This activation then phosphorylates many downstream targets, including 4E-BP1, S6K, and LARP1 [57]. Recent studies indicate that mTOR also modulates mitochondrial functions, stimulating the synthesis of nucleus-encoded mitochondria-related proteins such as mitochondrial ribosomal proteins, components of complexes I and V, and TFAM [58]. These signaling pathways that drive protein production require massive amounts of energy and are therefore called energy-consuming pathways. Compared to mTORC1, which is sensitive to acute rapamycin treatment, mTORC2 is only sensitive to prolonged treatment [59]. Currently, mTORC2 is known to phosphorylate Akt/protein kinase B, protein kinase C, and serum- and glucocorticoid (GC)-induced kinase, thereby being involved in actin reorganization and regulating metabolism, cell survival, and Na+ transport [60]. However, how much intracellular energy the mTORC2 pathway consumes is still unclear.
5.3.2 Energy-Economizing Process: Unfolded Protein Response (UPR) and Endoplasmic Reticulum Stress (ER Stress)
Many cellular events are orchestrated by antagonistic pathways or molecules to maintain cell survival and viability such as oxidation vs. antioxidation and apoptosis vs. survival. Together with active energy-consuming systems such as the mTORC1 pathway mentioned above, cells are equipped with so-called anti-energy-consuming pathways or energy-economizing pathways that halt protein production. During protein production, glycoproteins undergo special and precise processes to be transported to their final destination. Only accurately folded proteins are allowed to exit the endoplasmic reticulum (ER) and be transported to the Golgi compartment. Unfolded and misfolded proteins are either retained in the ER in a complex with molecular chaperones or degraded in a process referred to as ER-associated degradation [61]. When these immature proteins accumulate beyond the ER handling capacity, so-called ER stress occurs and promotes cell survival by arresting protein translation and stimulating the gene expression of molecular chaperones [62]. This signaling pathway linking ER imbalance to gene expression is named the unfolded protein response (UPR). The concept of UPR was initiated by the identification of two membrane proteins that were induced in avian sarcoma virus-transformed cells [63]. Induction of glucose-regulated proteins 78 and 98, referred to as GRP78 and GRP94, was not a direct effect of cellular transformation, but was secondary to the rapid depletion of glucose from the growth medium of rapidly growing sarcoma virus-transformed fibroblasts [64]. Further studies identified GRP78 (alternatively named BiP) as a representative ER-resident chaperone that is bound to polypeptides and regulates protein folding in the ER [65, 66]. In addition, other ER chaperones, including calreticulin, play crucial roles in the trimming of sugar bonds from glycoproteins and in correct folding. It is important to note that these ER chaperones successfully function in the presence of sufficient ATP. Therefore, the lack of ATP leads to the upregulation of ER chaperones, in which GRP78/BiP up-expression is a commonly used indicator to determine that cells undergo UPR/ER stress.
5.4 UPR/ER Stress and Glomerular Proteinuria
The involvement of UPR/ER stress in the pathogenesis of glomerular disease is a relatively new story [12, 67, 68]. The first report indicating the role of ER stress in glomerular proteinuria was demonstrated in Heymann nephritis, a rat model of human membranous nephropathy [69, 70]. This study revealed that GRP78 was upregulated and that both PERK and eIF2α, downstream UPR molecules, were activated in the glomeruli at the proteinuric stage. Subsequently, another group demonstrated a pathogenic role for the accumulation of misfolded proteins in the ER in glomerular podocytes in vivo. A transgenic rat overexpressing megsin, a member of the serine protease inhibitor family, developed massive proteinuria [71, 72]. Recently, Pierson syndrome, a congenital nephrotic syndrome with a mutation in the laminin β2 gene, was suggested as a prototypical ER storage disease. Authors generated transgenic mice carrying a mild laminin β2 missense mutation. This mutation caused defective secretion of laminin α5β2γ1 from the podocyte to the glomerular basement membrane (GBM). Interestingly, in this animal model ER stress preceded proteinuria and was still associated with proteinuric stage [73]. These in vivo models support the idea that excess protein accumulation in podocytes causes glomerular proteinuria through activating UPR/ER stress. Therefore, does ER stress also underlie the pathogenesis of proteinuria in acquired nephrotic syndrome? Puromycin aminonucleoside (PAN) nephrosis is a well-known rat model resembling minimal change nephrotic syndrome (MCNS) [74]. However, the pathogenesis of proteinuria has remained unclear. Previously, we revealed that glomeruli at the proteinuric stage of PAN nephrosis exhibited an upregulation of GRP78 mainly in the podocyte [40]. In vitro experiments further determined that energy depletion resulted in the accumulation of hypoglycosylated immature nephrin in the ER associated with UPR activation (GRP78 upregulation). Interestingly, the hypoglycosylation and mislocalization of nephrin were rescued by treatment with GC and mizoribine through the recovery of intracellular ATP [40, 75, 76]. These experiments led us to hypothesize that the arrested protein production of nephrin under ER stress was a main cause of proteinuria in PAN nephrosis.
5.5 mTORC1 Activation Upstream of UPR/ER Stress in Glomerular Proteinuria
As described so far, in the protein production system, mTORC1 accelerates production associated with energy consumption, whereas UPR halts the process to economize energy. Therefore, these two signaling networks have been understood as separate pathways that are involved in different cellular processes. However, recent studies, including ours, indicate that tight interconnections between mTORC1 and UPR activation also underlie many pathological events [77–80]. The first evidence showing the cross talk between UPR/ER stress and the chronic activation of mTORC1 emerged in 2008. It was revealed that loss of function of the tuberous sclerosis complex genes (TSC1 or TSC2) led to constitutive activation of the mTORC1 signaling pathway, resulting in the development of tumors and neurological disorders [77]. Interestingly, this loss of TSC1 or TSC2 also caused ER stress and activated UPR [77]. This was further demonstrated in a murine model of diabetic nephropathy (DN). DN is speculated to be a podocyte disease. However, the molecular mechanisms of podocyte dysfunction in the development of DN remain unclear. A study revealed that mTORC1 activity was enhanced in the podocytes of diabetic animals [81]. Podocyte-specific mTORC1 activation induced by ablation of an upstream negative regulator (PcKOTsc1) resulted in podocyte loss, GBM thickening, mesangial expansion, and proteinuria in nondiabetic young and adult mice [81]. In this model, abnormal mTORC1 activation induced the mislocalization of nephrin with enhanced ER stress in podocytes. 4-Phenyl butyric acid, a chemical chaperone that reduced UPR/ER stress, is protected against both the podocyte phenotypic switch and podocyte loss in PcKOTsc1 mice [81]. These previous studies indicate that mTORC1 activation is a critical step that leads to UPR/ER stress in podocytes, which may be a directly responsible event underlying the pathology of glomerular proteinuria.
Could be the same scenario as described in DN be adopted for the pathology of MCNS? One interesting paper showed that pretreatment with mTORI (everolimus) significantly protected against proteinuria in PAN nephrosis [82]. The authors, however, proposed that the protective effect of mTORI was due to a reduction in macrophage invasion and not a direct effect against podocyte injury. To identify the interconnection between mTORC1 activation and UPR/ER stress induction in this model, we consecutively analyzed the glomeruli between day 0 and day 5 after PAN injection [79]. Significant proteinuria was initiated on day 2 after PAN injection. In the control glomeruli from mice treated with saline, the majority of the GRP78 staining was observed in the cytoplasm of the podocyte cell bodies. In contrast, in day 1 glomeruli after PAN injection, which is 1 day prior to proteinuria, increased GRP78 was found in the podocytes, including the foot processes. On and after day 2, the GRP78 podocyte expression increased in association with the exacerbation of proteinuria. In the control glomeruli, nephrin was demonstrated as having a GBM pattern, indicating its proper localization at the slit membrane. In contrast, the overall distribution of nephrin in days 1 and 2 glomeruli consisted of a GBM pattern and a podocyte cytoplasmic pattern, and clear overlap of cytoplasmic nephrin with GRP78 was determined. These results indicated that some nephrin was retained in the podocyte cytoplasm. On days 3 and 5, GBM-pattern nephrin was drastically reduced and the majority of nephrin was cytoplasmic and colocalized with GRP78. In addition, in day 1 and day 2 glomeruli, low molecular weight, or immature, nephrin was observed, and nephrin mRNA was rather increased. These results suggested that UPR–ER stress occurred in podocytes and immature nephrin accumulated in the ER, leading to structural defects in the slit membrane and causing heavy glomerular proteinuria. With the studies using the sample from in vivo such as isolated glomeruli, it is difficult to conclusively distinguish the time lag between the rapid activation of the signaling pathways. In cultured podocyte experiments treated with PAN, mTORC1 activation actually preceded UPR activation [79]. In an in vivo model, PAN rats treated with mTORI (everolimus) clearly showed inhibition of proteinuria and also preserved nephrin expression. All the cellular signaling pathways demand energy that is driven by the hydrolysis of ATP to ADP and phosphate or AMP and pyrophosphate. The energy charge, which is defined as {[ATP] + §[ADP]}/{[ATP] + [ADP] + [AMP]}, reflects the cellular balance between the energy-consuming state and the energy-producing state [83]. The energy charge implies that AMP drives the cellular energy balance toward the energy-producing adaptation [84]. In our nephrotic syndrome model, AMPKα, a sensor of glomerular energy status, was activated after mTORC1 activation. In addition, the intracellular ATP levels in cultured podocytes decreased after mTORC1 activation, with a concurrent increase in the AMP levels, whereas the ADP levels showed no significant change. Overall, these changes represent a marked decrease in the energy charge [79], and this finding indicates that the cells appeared to be shifted to an energy-deficient state. Indeed, when PAN rats were treated with everolimus after PAN injection, proteinuria was not changed at all (unsubmitted data), conclusively indicating that mTORC1 activation is a primary cause of PAN nephrosis. Lipopolysaccharide (LPS) is also a well-known inducer of nephrotic syndrome in mice [85]. LPS activates a signaling pathway through interaction with toll-like receptor 4 [86, 87]. However, its mechanism of inducing proteinuria remains elusive. Interestingly, LPS activated mTORC1 in the glomerular podocytes during the proteinuric state [12]. We did not continuously evaluate the LPS-treated mice because the proteinuric phase of this model is very short, starting at 12 h and disappearing at 48 h after LPS injection [88]. Instead, cultured podocytes were treated with LPS and consecutively analyzed for changes in signaling activity. LPS treatment for 3 h activated phospho-70S6K, a downstream target of mTORC1, and AMPKα. These proteins remained active for 48 h [12].
In conclusion, mTORC1 activation seems to act upstream and secondarily induce UPR/ER stress in podocytes, thereby affecting the integrity of the podocyte filtration barrier and leading to heavy glomerular proteinuria.
5.6 mTOR Inhibitor Causes Glomerular Proteinuria
A great deal of mystery confounds the idea that mTORC1 activation triggers UPR/ER stress and leads to glomerular proteinuria. mTORI was originally used to replace calcineurin inhibitor to avoid nephrotoxicity and maintain kidney function in organ transplant patients [89, 90]. Subsequently, based on its antiproliferative and antiangiogenic effects, mTORI has also been used in the treatment of cancers such as renal cell carcinoma [91] and nonmalignant conditions such as autosomal dominant polycystic kidney disease [92]. However, mTORI causes a variety of adverse effects in many organs, including blood cells. Glomerular proteinuria is one of its well-known effects [93]. Exacerbated proteinuria occurs in up to approximately 40 % of kidney transplant patients, but the incidence of nephrotic syndrome is low (2 % for sirolimus) [93]. A randomized clinical study of autosomal dominant polycystic kidney disease revealed a significantly increased urinary albumin excretion rate in the sirolimus group compared to the control group, suggesting that the induction of sirolimus itself may cause de novo proteinuria. The mechanism by which mTORI causes proteinuria remains elusive. It has been reported that the combination of low-dose everolimus and low-dose CNI does not cause proteinuria in pediatric renal transplant patients [94, 95]. On the other hand, conversion of CNI to sirolimus revealed an apparent increase of proteinuria [96]. Thus, one hypothesis speculates that the elimination of the vasoconstrictive effects of CNI may underlie the pathogenesis of proteinuria. Reduction of vascular endothelial growth factor (VEGF) expression is also hypothesized as another cause of proteinuria. mTORI (rapamycin) exerts antiangiogenic effects linked to decreased VEGF production and also inhibits the response of vascular endothelial cells to VEGF stimulation [97, 98]. VEGF is prominently expressed in glomerular podocytes and in tubular epithelial cells in the kidney, while VEGF receptors are mainly found on preglomerular, glomerular, and peritubular endothelial cells [99]. Interestingly, mice heterozygous for VEGF-A in the podocyte exhibited endotheliosis and loss of endothelial fenestration, leading to nephrotic syndrome [100]. Presently, no definite evidence can explain a direct involvement of downregulation of mTOR and inactivation of mTORC1 signaling in the induction of UPR/ER stress. However, this idea is worth studying in the future.
5.7 Energy Metabolism in the Podocyte as a Therapeutic Target
Energy metabolism pathways, especially in mitochondria, have emerged as new therapeutic targets in many intractable diseases, including kidney diseases [101–103]. Over the past five decades, synthetic GCs have been employed as a first-line treatment for patients with acquired nephrotic syndrome. Despite their obvious clinical effect on nephrotic syndrome, especially with the minimal change patients, how GCs improve heavy proteinuria is largely unknown. The pharmacological action of GCs is executed principally through binding to a cytosolic receptor, the glucocorticoid receptor (GR) [104, 105]. Although blood lymphocytes are the most likely GC therapeutic target, the injured glomerular podocytes are also considered to undergo GC effects. In 1999, we discovered that all human glomerular cells possess GR both in the cytoplasm and in the nucleus, where GR is dynamically translocated between both compartments [106]. We additionally revealed that the glucocorticoid-inactivating enzyme 11β-hydroxysteroid dehydrogenase type 2 functions in human glomerular podocytes [107]. Thus, we proposed that GCs may directly act on the injured glomerular podocytes and that GCs are metabolized in these highly differentiated cells. We also showed that the mitochondria in glomerular podocytes expressed the GR and that GC stimulated ATP production through the upregulation of mitochondrial genes [76]. The protective effect of GCs on ER-stressed cells was obvious in our in vitro nephrotic syndrome model. However, there is no doubt that GCs possess pharmacological functions with more effects on the energy regulatory machinery than those that we have proposed thus far. In conclusion, a better understanding of energy metabolism in activated and quiescent podocytes will provide insights into the elucidation of many pathologies and the development of future therapeutic interventions.
< div class='tao-gold-member'>
Only gold members can continue reading. Log In or Register a > to continue
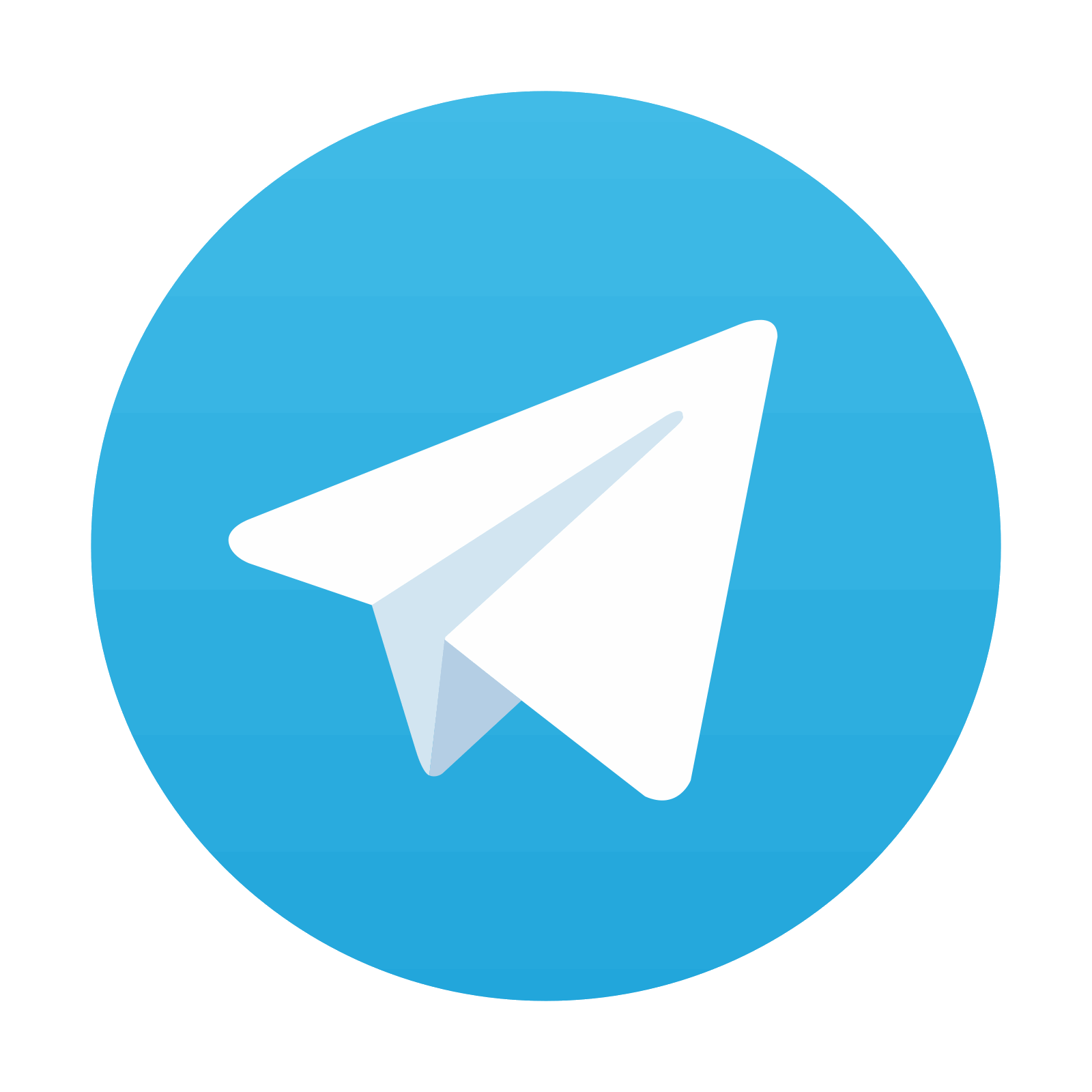
Stay updated, free articles. Join our Telegram channel
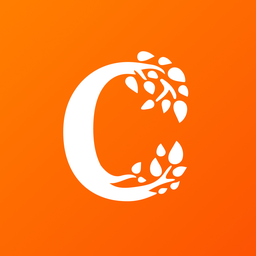
Full access? Get Clinical Tree
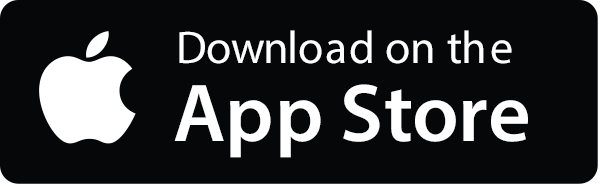
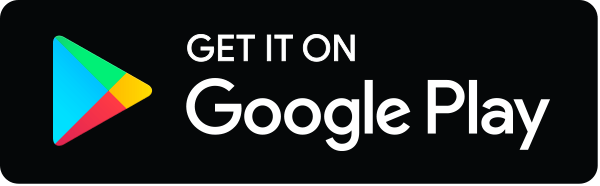