Fig. 17.1
The B-mode image (right panel) and the elastogram (color) overlaid on B-mode image (left panel) were displayed side by side. Lower part P1, middle part P2, upper part P3 (about one-third each) and the cervical channel. The strain ratio (left ) in P1 is higher than the strain rate (right) in P3, which corresponds to the color distribution. (From Khalil et al. [15], with permission)
In the study conducted by Swiatkowska-Freund et al., 29 women who underwent term labor induction were evaluated by elastography. The cervix was scanned while being mildly compressed with the ultrasound probe. They reported a significant difference in mean elastography index of the internal os between the patients with successful induction and those with failed induction. On the other hand, tissues near the external os and the middle of the canal did not show similar trends. The authors observed that elastography “may guide decisions about need for cervical ripening before labor induction,” noting that “the technology is limited without standardization between subjects” [16, 17]. In a cross-sectional study of 112 pregnant women at all points in gestation, no statistically significant difference between cervices was found. From the noticeable strain readings in the area where the compression was directly received, the authors addressed concerns that “measurements of rate-of-change in tissue displacement” may be “a mere reflection of the force being applied by the transducer” and might not “reflect histological changes that could provide a measure of cervical ripening.” A serious step has to be taken for standardizing compression procedures that can vary between scans and subjects before elastography can be a reliable clinical tool [17, 18]. It also should be noted that the underlying structures of women’s reproductive systems are complex, making it more challenging to apply pressure in a standardized way.
Acoustic Radiation Force Impulse and Shear Wave Elasticity Imaging (ARFI and SWEI) of the Cervix and Uterus
An operator-independent method that does not require standardization would be ideal. Instead of applying compression using an ultrasound probe, acoustic radiation force due to focused ultrasound can be used for remote and controlled palpation: (1) monitoring the tissue response in displacement within the radiation force region of excitation (ROE) and generating images of relative differences in tissue stiffness (acoustic radiation force impulse [ARFI] imaging ); and (2) monitoring the speed of shear wave propagation away from the ROE to quantify tissue stiffness (shear wave elasticity imaging [SWEI ]). In response to electronically controlled acoustic radiation force, the resulting tissue displacement and the shear wave propagation speed directly reflect stiffness of the underlying tissue [19]. The ability of SWEI to classify ripened versus unripened tissue samples was assessed by Carson et al. using excised human hysterectomy samples. The authors stated that “if the location is accounted for, comparisons between patients can distinguish between ripened versus unripened subjects” [20].
In their human subject study, Su et al. applied ARFI and SWEI ultrasound imaging of the uterine cervix on 58 patients with pathologically confirmed cervical cancer prior to surgery. They found a statistically significant difference between the malignant lesions (stiff) and normal cervical tissues (soft). With relatively high sensitivity and specificity in the evaluation of cervical cancer, the authors stated that ARFI or SWEI can be an objective method for stiffness assessment and “may have good diagnostic value in clinical applications” [21]. In a case study of two subjects with strongly suspected leiomyosarcoma and leiomyoma, Furukawa et al. reported that SWEI can be a useful method for diagnosing uterine smooth muscle tumors (Fig. 17.2) [22].


Fig. 17.2
Ultrasound images of uterine leiomyosarcoma (a) and leiomyoma (b). Left: Ultrasound B-mode image. Right: Acoustic radiation force impulse (ARFI) image. Irregular distribution of blue, yellow, green, and red was seen in the ARFI image, suggesting a heterogeneous inner structure. Notable blue was present in high echoic spots shown on gray-scale imaging. (From Furukawa et al. [22], with permission)
Gennisson et al. applied SWEI on the cervix and uterus of women during pregnancy, which enabled the quantification of cervix elasticity, the follow-up of uterine elasticity during contraction, and the investigation of uterine anisotropy. In their study, cervix elasticity was quantified in 20 gravid women using a 7 MHz endocavitary probe. Uterus elasticity was quantified externally on 5 patients, through the abdomen, using an 8 MHz linear probe. Changes of elasticity were monitored in real time during uterus contraction (Fig. 17.3). SWEI was performed with the same probe by assessing shear wave speed variations with respect to probe angle, which allowed the investigation of uterine anisotropy at different depths. Elasticity values during contraction were correlated to a gold standard of uterine pressure measure [23].


Fig. 17.3
Snapshot of the quantified elasticity map of the uterus during contraction. (From Gennisson et al. [23], with permission)
In the study conducted by Tanaka et al., to investigate whether baseline stiffness of the uterine corpus and cervix changed after placental delivery, SWEI was applied on 11 patients with normal vaginal delivery before, immediately after, and 1 and 2 h after placental delivery. It was found that “the stiffness of the uterine corpus significantly changed over time, although that of the uterine cervix was not significantly altered.” The authors also reported that (1) “The stiffness of the uterine corpus was significantly higher immediately after and 1 and 2 h after placental delivery as compared with that before placental delivery,” and (2) “The uterine corpus had a significantly higher stiffness than the uterine cervix at each of the four time points examined” [24]. While some effects due to acoustic attenuation at depth are naturally expected, Hernandez-Andrade et al. demonstrated that depth from the ultrasound probe to different regions in the cervix did not significantly affect the shear wave speed estimations in the cervix of 154 pregnant women at 11–36 weeks of gestation [25].
Using tissue motion tracking methods, some important information associated with dynamic responses of the pelvic floor muscles (PFM ) to potentially incontinence-producing stress, which cannot be readily captured and assimilated by the observer during the scanning process, was assessed by Peng et al. In their study, perineal ultrasonography was performed on 22 asymptomatic females and nine stress urinary incontinent (SUI) patients with a broad age distribution and parity. The ventral dorsal and cephalad-caudal movements of the anorectal angle were resolved, and kinematic parameters, in terms of displacement, trajectory, velocity, and acceleration were analyzed. The results revealed the possible mechanisms of PFM responses to prevent the urine from incontinence in fast and stress events. The statistical analyses showed the PFM responses of the healthy subjects and the SUI patients are significantly different in both the supine and standing experiments [26].
Photoacoustic Imaging of Ovarian Tissue and the Cervical Canal
Photoacoustic (PA) technology is the promising state-of-the-art medical imaging modality to provide a non-invasive quantitative optical contrast, at depth, and in real-time by using energy conversion from absorbed light energy to acoustic wave in tissue [27–29]. Salehi et al. used a hand-held transvaginal probe they designed for co-registered photoacoustic and ultrasound imaging to image ex vivo benign and malignant human ovaries (Fig. 17.4). They were able to produce co-registered images that displayed different vasculature distributions on the surface of the benign cyst and the malignant ovary [30, 31].


Fig. 17.4
Co-registered image of ovary 3. (a) Ultrasound-only image. (b) Photoacoustic image on top of the ultrasound image. (c) Hematoxylin and eosin (H&E) stained histological slide. The white bar represents 5 mm. (From Aguirre et al. [32], with permission)
Using their 1.75-D array transducer , Aguirre et al. imaged normal porcine ovarian tissue as compared to histological images (see Fig. 17.4). The authors stated that their results showed excellent co-registration of ultrasound and photoacoustic images. They described strong optical absorption from vasculature, especially highly vascularized corpus luteum and low absorption from follicles [32].
Photoacoustic imaging (PAI) is sensitive to the abnormal angiogenesis deep in biological tissue, and may be capable of intact scanning both around the external orifice and in cervical canal. With this hypothesis, Peng et al. applied PAI on 30 human tissue sample harvested from the cervical canal by biopsy during a cervical colposcopical screening. They reported stronger absorption from the cervical lesions compared to normal tissue. The estimated mean optical absorption from PAI between normal tissue and cervical lesion exhibited statistically significant difference [33].
Using cellulose-based nanoparticles with a peak photoacoustic signal of 700 nm, photoacoustic imaging was applied on a mouse model of human ovarian cancer (Fig. 17.5). An obvious increase of photoacoustic signal intensity in the tumor was observed when the pre-injection images (Panels B-D) were compared to the post-injection (Panels Bi-Di). Of note, the cellulose-based nanoparticles were shown to biodegrade in the presence of cellulose, a naturally occurring enzyme, indicating important advantages for clinical translation [34].


Fig. 17.5
Photoacoustic imaging of mouse tumor. Panels A and Ai are two different views of the imaging plane used to create the renderings in panels B–D (dashed lines). The top images are before injection of carbon-based nanopipettes (CNPs) and lower panels denoted by “i” are post-injection. B, no injection; C, 1.2 mg/mL; and D, 2.4 mg/mL. Intensity bar in D applies to all images, as does the scale bar in Di, which represents 3 mm. Arrows highlight regions with particularly increased photoacoustic contrast in post-injection images. (From Jokerst et al. [34], with permission)
Tactile Imaging of the Female Pelvic Floor
At the end of the last century, a technology named elastography , or elasticity imaging (EI) , for measuring and visualizing soft tissue viscoelastic characteristics, emerged and the ancient art of palpation gained new life [35]. The areas of applications of EI in medical diagnostics and treatment monitoring are steadily expanding. It has been shown that the elasticity of soft tissue is highly sensitive to its structure and conditions. The range of variation of the Young’s modulus of soft tissues is over three orders of magnitude, from a fraction of kPa to hundreds of kPa and appears to be one of the most sensitive physical characteristics of soft tissue, yielding valuable diagnostic information [5, 36]. Many pelvic floor disorders, including prolapse, SUI, sexual dysfunction, congenital anomalies, and others, are clearly manifested in the mechanical properties of pelvic structures. Therefore, the ability of EI to map elasticity of pelvic floor opens new possibilities in the biomechanical assessment and monitoring of pelvic floor conditions [37, 38]. EI technique based on tactile imaging additionally allows measuring muscle contraction capability.
Tactile imaging (TI), also called “mechanical imaging ,” is a medical imaging modality that translates the sense of touch into a digital image. The tactile image is a function of P(x,y,z), where P is the pressure on soft tissue surface under applied deformation, and x,y,z are coordinates where pressure P was measured [39, 40]. The tactile image is a pressure map on which the direction of tissue deformation must be specified [40].
Functional Tactile Imaging
Functional tactile imaging (FTI) is a variation of TI that translates muscle activity into a dynamic pressure pattern P(x,y,t) for an area of interest, where t is time and x,y are coordinates where pressure P was measured. Muscle activity to be studied may include a voluntary contraction (e.g., a pelvic floor squeeze), an involuntary reflex contraction (e.g., due to a cough), an involuntary relaxation, or a Valsalva (veering down) maneuver [41, 42].
A vaginal tactile imaging (VTI) probe , as shown in Fig. 17.6 , is equipped with 96 pressure (tactile) sensors laid out at every 2.5 mm along the both sides of the probe, an orientation sensor (accelerometer), and temperature sensors with micro-heaters [43]. During the clinical procedure, the probe is used to acquire pressure responses from the vaginal walls. The VTI examination procedure includes data collection from all segments of the vagina. During an examination, data are sampled from the probe sensors and displayed on the VTI monitor in real time. The resulting pressure maps (tactile images) of the vagina integrate all of the acquired pressure and positioning data for each of the pressure-sensing elements. In addition, the VTI records the dynamic contraction for pelvic floor muscles.
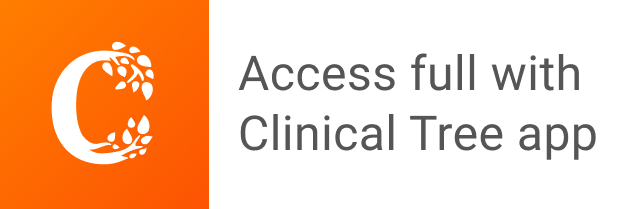