Chlorothiazide, which became available in 1958, ushered in the modern era of diuretic therapy, initially for the treatment of edematous states and shortly thereafter for the treatment of essential hypertension. Over the ensuing 60 years, diuretics have emerged as important therapeutic tools. First, they are capable of reducing blood pressure (BP), while simultaneously decreasing the morbidity and mortality that attends the poorly treated hypertensive state. Diuretics are currently considered as one of several first-line therapies suggested by various guideline-promulgating committees. In addition, they remain a necessary treatment tool for volume overload states, such as nephrotic syndrome and heart failure (HF), in that they afford important decongestion benefits. This chapter reviews the various diuretic classes and the physiologic adaptations that ensue from their use and establishes the basis for their use in the treatment of volume overload and various types of hypertension.
Individual Classes of Diuretics
The predominant nephron sites of action of the various diuretic classes are represented in Fig. 9.1 . Inter- and intraclass differences exist for all diuretic groups. The diuretic classes of note include proximal tubular, distal convoluted tubule (DCT) and loop diuretics, potassium (K + )-sparing agents, and osmotic diuretics.

Proximal Tubular Diuretics
The administration of a carbonic anhydrase (CA) inhibitor ordinarily results in a brisk alkaline diuresis. By inhibiting CA, these compounds decrease the generation of intracellular H + , which is a prerequisite for the absorption of sodium (Na + ); therein resides their primary diuretic action (see Fig. 9.1 ). Although CA inhibitors work at the proximal tubule (PT) level where the bulk of Na + reabsorption occurs, their final diuretic effect is lessened by reabsorption in more distal nephron segments. Acetazolamide is currently the only CA inhibitor used mainly for its diuretic properties; other CA inhibitors are used topically in the treatment of glaucoma. Acetazolamide is readily absorbed and is eliminated by tubular secretion (≈50%). Its use is constrained by its transient action and because prolonged use results in a metabolic acidosis, among other side effects. Alternatively, acetazolamide (250 to 500 mg daily) corrects the metabolic alkalosis that can occur with thiazide or loop diuretic therapy. Acetazolamide should be used cautiously in patients with advanced kidney failure because it systemically accumulates with repeat dosing with resultant neurologic side effects. In addition, acetazolamide may reduce the glomerular filtration rate (GFR) when given intravenously; however, the dose dependency of this process is poorly understood. The anticonvulsant topiramate inhibits CA and therein can cause metabolic acidosis. Patients with a history of renal calculi or known renal tubular acidosis should receive topiramate with some caution, if at all, unless other treatment options are not available.
Distal Convoluted Tubule Diuretics
The major site of action of thiazide diuretics is the early DCT where they inhibit the coupled reabsorption of Na + and chloride (Cl − ). The water-soluble thiazides such as hydrochlorothiazide (HCTZ) also inhibit CA when given at high doses, and therein further increase Na + excretion. Thiazides also inhibit NaCl/fluid reabsorption in the medullary-collecting duct. In addition to these varied effects on Na + excretion, thiazide diuretics impair urinary diluting capacity without affecting urinary concentrating mechanisms, reduce calcium (Ca ++ ) and urate excretion, and increase magnesium (Mg ++ ) excretion. The most widely prescribed drug in this class is HCTZ. The onset of diuresis with HCTZ occurs within 2 hours, peaks between 3 and 6 hours, and dose-dependently continues for upward to 12 hours. The half-life ( ) of HCTZ is prolonged both in decompensated HF and kidney failure. Doses of thiazide diuretics in the 100 to 200 mg/day range are required to initiate a diuresis in patients with chronic kidney disease (CKD), although the overall natriuretic response is arbitrated by the GFR/filtered Na + load.
The concept of “class effect” is debated relative to the actions of DCT diuretics, as applied to both BP reduction and cardiovascular (CV) outcomes. Much of the recent debate on diuretic class effect has centered on the differences between chlorthalidone and HCTZ. Although chlorthalidone and HCTZ are structurally similar, they differ pharmacokinetically in that chlorthalidone has a longer of 40 to 60 hours versus 3.2 to 13.1 hours for HCTZ, as well as a larger volume of distribution by virtue of its extensive partitioning into red blood cells. This latter feature creates a depot that promotes continuous streaming of chlorthalidone (red cell → plasma → tubular secretion). This plasma half-life difference for chlorthalidone correlates with a more extended diuretic effect and is a likely explanation for the observation that chlorthalidone is a better mg-for-mg antihypertensive than HCTZ.
Loop Diuretics
Loop diuretics act predominantly at the luminal membrane in the thick ascending limb (TAL) of the loop of Henle where they compete with Cl − for binding to the Na + /K + /2Cl − cotransporter, thereby inhibiting Na + and Cl − reabsorption. Loop diuretics also have qualitatively minor effects on Na + reabsorption within other nephron segments. Other clinically relevant effects of loop diuretics include a decrease in both free water (H 2 O) excretion and absorption during H 2 O loading and dehydration, respectively, a 30% increase in fractional Ca ++ excretion, a significant increase in Mg ++ excretion, as well as a brief increase followed by a more long-lived decrease in uric acid excretion. Loop diuretics also stimulate renal prostaglandin synthesis, particularly that of the vasodilatory prostaglandin E 2 (PGE 2 ). Angiotensin-II, generated following the administration of intravenous loop diuretics, coupled with an increased synthesis of PGE 2 , are the likely reasons for the shift in renal blood flow (RBF) from the inner to the outer renal cortex with these drugs; however, both total RBF and GFR are maintained when loop diuretics are administered to normal subjects.
The available loop diuretics include bumetanide, ethacrynic acid, furosemide, and torsemide. These compounds are highly protein bound, mainly to albumin; therefore to gain access to their tubular site of action they must be secreted as is the case for thiazide diuretics. This process occurs via probenecid-sensitive organic anion transporters localized to the PT. Tubular secretion of loop diuretics may be interfered by elevated levels of endogenous organic acids, as arise in CKD, and by drugs that share the same transporter site, such as salicylates and nonsteroidal antiinflammatory drugs (NSAIDs). Uremic toxins and fatty acids can decrease loop diuretic protein binding and further alter diuretic pharmacokinetics.
Diuretic excretion rates approximate drug delivery to the medullary TAL and correlate with the observed natriuretic response. The relationship between the urinary loop diuretic excretion rate and natriuresis is that of an S -shaped sigmoidal curve. A normal dose-response relationship (as is typically seen in the untreated patient with hypertension) can be shifted (downward and rightward) by a variety of clinical conditions ranging from volume depletion (“braking phenomenon”) to HF or nephrotic syndrome (disease-state alterations) to various drug therapies. As an example of the latter, NSAIDs modify this dose-response relationship by inhibiting prostaglandin synthesis and blunting an expected diuretic effect. Finally, the binding of loop diuretics to urinary protein seems not to be the basis for the blunted diuretic effect in the setting of nephrotic syndrome as was suggested early on.
Furosemide is the most widely used diuretic in this class; however, its use is complicated by variable absorption with a bioavailability ranging from 12% to 112%. The coefficient of variation for absorption varies from 25% to 43% for different furosemide products, and exchanging one furosemide formulation for another will not standardize patient absorption and/or response to oral furosemide. Bumetanide and torsemide are better absorbed than is furosemide. The consistency of torsemide’s absorption and its longer duration of action are features to consider when loop diuretic therapy is needed in the chronic HF patient. Loop diuretics are commonly used in the patient with CKD, with the renal clearance of these drugs reduced in parallel with the degree of reduction in kidney function. In general, furosemide pharmacokinetics are more significantly changed in CKD than the other loop diuretics, with both its renal metabolism and intact clearance reduced in CKD. Alternatively, bumetanide and torsemide undergo significant hepatic metabolism, which is only marginally impacted by CKD, and in CKD their pharmacokinetic profiles only change as the result of decreased renal clearance of the intact molecules.
Distal Potassium-Sparing Diuretics
There are two classes of K + -sparing diuretics: competitive antagonists of aldosterone, such as spironolactone or eplerenone, and compounds that work independent of aldosterone, such as amiloride and triamterene. Drugs in this class reduce active Na + absorption in the late DCT/collecting duct (CD). In so doing, basolateral Na + /K + -ATPase activity falls off, intracellular K + concentration decreases, and the electrochemical gradient for K + is lowered, thereby reducing K + secretion. K + -sparing diuretics also reduce Ca ++ and Mg ++ excretion, which is a useful feature in the HF patient. Because K + -sparing diuretics are only modestly natriuretic, their clinical utility resides more in their K + -sparing capacity, particularly when more proximally acting diuretics increase distal Na + delivery or in the instance of either primary or secondary aldosteronism.
Spironolactone is a well-absorbed, highly protein-bound, lipid-soluble K + -sparing diuretic with a 20-hour half-life. The onset of action for spironolactone is characteristically slow, with a peak response at times 48-hours or more after the initial dose. 7α-thiomethylspirolactone and canrenone are the two main metabolites of spironolactone that account for a good portion of its mineralocorticoid receptor blocking activity. Spironolactone, unlike amiloride and triamterene, remains active (as a diuretic and antihypertensive agent) in advanced kidney failure in that its locus of action is basolateral; thus it does not require glomerular filtration or tubular secretion to gain access to its effect site. Eplerenone is a mineralocorticoid receptor antagonist that is highly selective for the aldosterone receptor; accordingly, its much lower affinity for androgen and progesterone receptors results in considerably less gynecomastia than is the case with spironolactone. Typically, eplerenone is at best a very mild diuretic, and its antihypertensive effects originate from nondiuretic aspects of its action.
Amiloride and triamterene are K + -sparing diuretics that block epithelial Na + channels (ENaC) in the luminal membrane of the CD. They are both actively secreted by cationic transporters that reside in the PT, and each has but a very modest natriuretic effect. The cationic nature of these compounds is such that they interfere with the tubular secretion of creatinine. Both drugs are seldom used in HF for diuresis; rather, they may be used for their K + – and Mg ++ -sparing properties. Amiloride and triamterene are both extensively renally cleared and will accumulate with repetitive dosing in the setting of a reduced GFR.
Osmotic Diuretics
Mannitol is a polysaccharide diuretic given intravenously that is freely eliminated by glomerular filtration. Mannitol is poorly reabsorbed along the length of the nephron and thereby exerts a dose-dependent osmotic effect. This osmotic effect traps water and solutes in the tubular fluid, thus increasing Na + , K + , Cl − , and excretion. The plasma
of mannitol depends on the level of kidney function but usually is between 30 and 60 minutes; thus its diuretic properties are quite transient. Mannitol has been used to reduce the incidence of acute kidney injury (AKI) in patients undergoing cardiopulmonary bypass, having rhabdomyolysis, or following exposure to contrast media. The findings from studies of these circumstances do not support a more widespread use of mannitol to prophylactically forestall development of AKI. Because mannitol also expands extracellular fluid (ECF) volume and can precipitate pulmonary edema in patients with HF, it should be used cautiously, if at all, in these patients. Moreover, excessive mannitol administration, particularly in the setting of a reduced GFR, can cause dilutional hyponatremia, hyperkalemia, and/or AKI. The latter is dose-dependent, relates to afferent arteriolar vasoconstriction, and commonly corrects with the elimination of excess mannitol as may be accomplished with hemodialysis.
Adaptation to Diuretic Therapy
Diuretic-induced inhibition of Na + reabsorption in one nephron segment elicits important adaptations in other nephron segments, which not only limits their antihypertensive and fluid-depleting actions but also contributes to the development of side effects. Although a portion of this resistance to diuretic effect is a normal consequence of diuretic use, disease-state related diuretic resistance is often encountered in patients with clinical disorders such as HF, cirrhosis, and kidney failure states marked by proteinuria.
The initial dose of a diuretic normally produces a brisk diuresis, which is quickly followed by a new equilibrium state in which daily fluid and electrolyte excretion either matches or is less than intake with body weight stabilizing. In nonedematous patients given either a thiazide or a loop diuretic, this adaptation, or braking phenomenon , occurs within 1 to 2 days and limits net weight loss to 1 to 2 kg. This braking phenomenon is most evident in normal subjects given a loop diuretic. For example, furosemide administered orally to subjects ingesting a high-Na + diet (270 mmol/24 hours) produced an initial brisk natriuresis, which resulted in a negative Na + balance over the ensuing 6 hours. This was followed by an 18-hour period when Na + excretion was reduced to levels well below the prescribed Na + intake, resulting in a positive Na + balance. This postdiuresis Na + retention matched the initial natriuresis with the result at the end of the day being a neutral Na + balance state and no weight loss. After 3 successive days of furosemide administration, a similar pattern of Na + loss and retention was demonstrated each day ( Fig. 9.2 ). This phenomenon is quite reproducible, being evident after even a month of furosemide administration. However, if Na + intake is kept very low, balance can remain negative after a single dose of furosemide, even though there is some blunting of the initial natriuretic response.

The mechanistic basis for the braking phenomenon is complex. The relationship between natriuresis and the rate of furosemide excretion is shifted to the right in subjects receiving a low-salt diet, which denotes a blunting of tubular response. The importance of ECF volume depletion in postdiuretic Na + retention has been clearly shown, although there is an ECF volume-independent component to this process as well. The latter appears to be unrelated to aldosterone, as spironolactone therapy has little effect on the Na + retention. Structural hypertrophy in the distal nephron also occurs in rats receiving prolonged infusions of loop diuretics. These structural changes are marked by increased distal nephron Na + and Cl − absorption and K + secretion, phenomena which are aldosterone independent. These structural adaptations may contribute to postdiuretic Na + retention and to diuretic tolerance in humans, and could explain the Na + retention persisting for up to 2 weeks after loop diuretic therapy is discontinued.
Neurohumoral Response to Diuretics
Plasma renin activity (PRA) and plasma aldosterone concentrations rise within minutes of receiving an intravenous diuretic, a process that is independent of volume loss and/or sympathetic nervous system (SNS) activation. This rise in PRA is caused by inhibition of NaCl reabsorption at the macula densa in conjunction with loop-diuretic stimulation of renal prostaglandin release. This first wave of neurohumoral effects, although transient, recognizably increases afterload and for a short period of time may lessen the efficacy of a loop diuretic. Shortly after this initial rise in PRA, diuretics cause a more sustained increase in PRA and aldosterone arising from an increase in SNS activity (β-agonism) and a fall in ECF volume. The increase in renal prostaglandin production is the likely explanation for the preload reduction and decrease in ventricular filling pressures that occur within 15 minutes of loop diuretic administration.
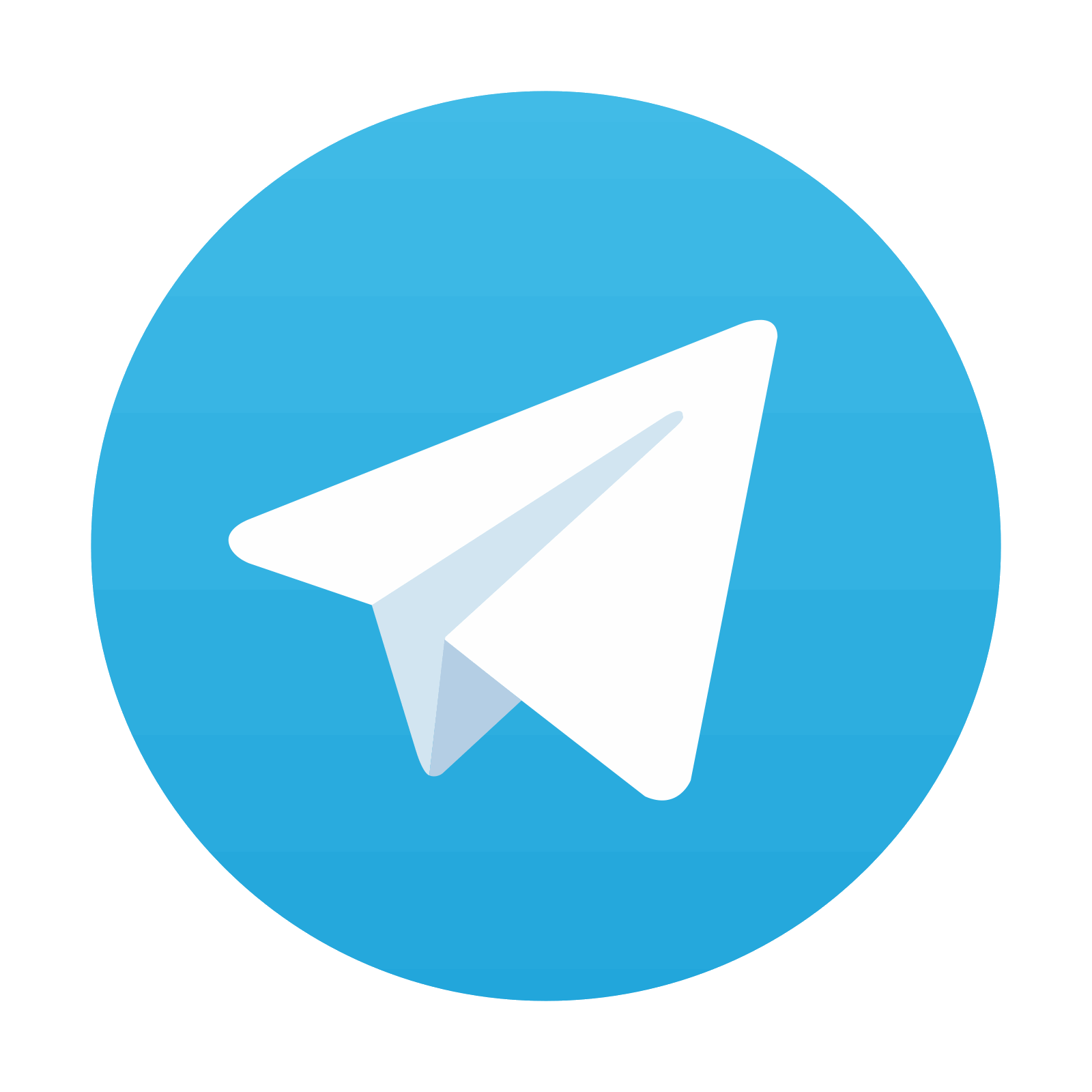
Stay updated, free articles. Join our Telegram channel
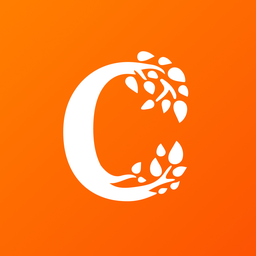
Full access? Get Clinical Tree
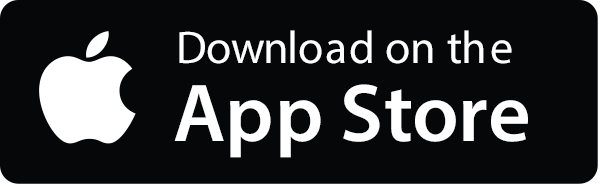
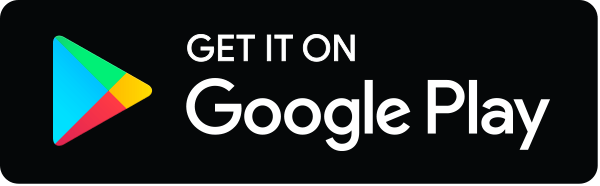