Abstract
Vitamin A is an essential nutrient for vision and life of humans because it is converted to the visual chromophore, 11- cis -retinal, and to the hormone, retinoic acid. Vitamin A in animal-derived foods is found as long-chain acyl esters of retinol and these are digested to free fatty acids and retinol before uptake by the intestinal mucosal cell. The retinol is then (1) reesterified to retinyl esters for incorporation into chylomicrons along with other dietary lipids and absorbed via the lymphatics or (2) effluxed into the portal circulation facilitated by the lipid transporter, ABCA1. Provitamin A carotenoids such as β-carotene (β-C) are found in plant-derived foods. These and other carotenoids that do not have vitamin A activity are transported into the mucosal cell by scavenger receptor class B type I (SR-BI). Provitamin A carotenoids are partly converted to retinol by oxygenase and reductase enzymes and the retinol so produced is available for absorption via the two pathways described. Carotenoids that are not converted to vitamin A are incorporated into chylomicrons for absorption. The efficiency of vitamin A and carotenoid intestinal absorption is determined by the regulation of a number of proteins involved in the process. Polymorphisms in these genes lead to variability in the metabolism and transport of vitamin A and carotenoids among individuals.
Keywords
Retinoid, Membrane transport, Lipase, Carotene oxygenase, Acyltransferase, Lipid, Chylomicron, Metabolism, Single nucleotide polymorphisms, Bioavailability
Abbreviations
ARAT
acyl-CoA:retinol acyltransferase
BCO1
β-carotene 15,15′-oxygenase
BCO2
β-carotene 9′10′-oxygenase 2
CEL KO
CEL knockout
CEL
carboxyl ester lipase
CM
chylomicrons
CRBP
cellular retinol-binding protein
DGAT
diacylglycerol acyltransferase
KO
knock out
LRAT
lecithin:retinol acyltransferase
LUT
lutein
LYC
lycopene
OA
oleic acid
PLRP
pancreatic lipase-related protein
PTL
pancreatic triglyceride lipase
RA
retinoic acid
RAR
retinoic acid receptors
RE
retinyl esters
REH
retinyl ester hydrolase
RPE
retinal pigment epithelium
RXR
retinoid X receptors
TC
taurocholate
TG
triglycerides
VLDL
very low-density lipoproteins
WT
wild type
ZEA
zeaxanthin
α-C
α-carotene
β-C
β-carotene
50.1
Introduction
Vitamin A deficiency affects more than 100 million children throughout the world. Thus, knowledge about the mechanisms of absorption of vitamin A can lead to better approaches for enhancing its absorption and could be helpful in ameliorating some of the deficiencies. The major sources of vitamin A in human diet are the provitamin A carotenoids in fruits and vegetables and retinyl esters found in foods of animal origin. In humans, carotenoids are either cleaved to generate retinol or absorbed intact. In contrast, retinyl esters are completely hydrolyzed in the intestinal lumen and free retinol is taken up by enterocytes. The intestinal absorption and metabolism of dietary carotenoids has been the subject of recent reviews, as have the biochemical and molecular mechanisms involved in the digestion and absorption of vitamin A.
50.2
Carotenoid and Vitamin A Metabolism—Overview
Carotenoids are synthesized in plants and in certain microorganisms such as some bacteria, algae, and fungi. They are a group of pigments that are widespread in nature and responsible for the yellow/orange/red/purple colors of many fruits, flowers, birds, insects, and marine animals. Over 600 carotenoids have been isolated from natural sources, but only ~ 60 of them are detected in the human diet and ~ 20 of them in human blood and tissues. β-Carotene (β-C), α-carotene (α-C), lycopene (LYC), lutein (LUT), and β-cryptoxanthin are the five most prominent carotenoids present in the human body.
All carotenoids are derived from the basic linear polyisoprenoid structure of LYC that contains 40 carbon atoms and an extended system of 13 conjugated double bonds. Carotenoids derive from this parent structure by cyclization (i.e., formation of β- or ε- ionone rings) at one (i.e., γ-carotene) or two ends (i.e., β-C and α-C) of the polyene chain and by dehydrogenation and/or oxidation. The structures of several major carotenoids are shown in Fig. 50.1 . The carotenoid group is divided into the carotenes, hydrocarbon carotenoids with unsubstituted rings, and xanthophylls, carotenoids with at least one oxygen atom. They exist mostly in the all- trans configuration, but they can be subject to a cis isomerization at any double bond of their polyene chain, resulting in a large number of mono- and poly- cis isomers.

Capability for the de novo synthesis of compounds with vitamin A activity is limited to plants and microorganisms. Thus, higher animals must obtain vitamin A from the diet, either as the preformed vitamin or as a provitamin carotenoid such as β-C. In the intestinal mucosa β-C is converted to retinal by β-C 15,15′ oxygenase 1 (BCO1) and the retinal is then reduced to retinol by a retinal reductase. In the human intestine about half the dietary provitamin A carotenoids are converted to retinol and about half are absorbed intact although the extent of conversion varies widely among individuals. The major dietary forms of preformed vitamin A are long-chain fatty acid esters of retinol. These esters must be hydrolyzed prior to intestinal absorption. Hydrolysis of the esters is catalyzed both by enzymes secreted by the pancreas into the intestinal lumen and by those associated directly with intestinal cells.
Following the hydrolysis of dietary retinyl esters, the free retinol is then taken up by the mucosal cell. The free retinol, resulting either from hydrolysis of dietary retinyl esters or by conversion of dietary provitamin A carotenoids, is reesterified with long-chain, mainly saturated, fatty acids by the enzyme lecithin:retinol acyltransferase (LRAT) when physiological doses of vitamin A are ingested. The resulting retinyl esters are incorporated with other neutral lipid esters (i.e., triacylglycerols and cholesteryl esters) and intact carotenoids into chylomicrons and absorbed via the lymphatics. Thus, co-ingestion of lipid to insure robust chylomicron formation is required for the efficient intestinal absorption of dietary carotenoids and vitamin A. In the vascular compartment, much of the chylomicron triacylglycerol is hydrolyzed by lipoprotein lipase in extrahepatic tissues resulting in the production of a “chylomicron remnant” that contains most of the newly absorbed retinyl esters. In the rat, the chylomicron remnants are rapidly and almost quantitatively taken up by the liver, and there is evidence that the retinyl esters are rapidly hydrolyzed and reesterified during this process.
Under conditions of adequate vitamin A nutriture, the liver is the main site of vitamin A storage, with over 95% of the total neutral retinoid being present as retinyl esters, predominately retinyl palmitate, and stearate. Although chylomicron remnants (and the retinyl esters they contain) are initially taken up exclusively by the hepatocytes in liver, the retinyl esters are then transferred largely to the perisinusoidal stellate cells. In vitamin A-adequate rats, the stellate cells account for approximately 80% of the total retinyl ester store with the remainder being in hepatocytes. In both cell types, the retinyl esters are stored in cytoplasmic lipid droplets along with other neutral lipids. Prior to mobilization from the liver the retinyl esters are hydrolyzed, and free retinol is complexed to serum retinol-binding protein for secretion from the liver. Unesterified retinol is delivered to peripheral tissues via RBP4 (serum retinol-binding protein), where it is converted to its two major biologically active metabolites, 11- cis -retinal and all- trans -retinoic acid (RA). The former is the chromophore for the visual pigment rhodopsin, and thus is essential in the visual cycle. The RAs (all- trans and 9- cis ) are the ligands for two families of hormone-dependent transcription factors, the retinoic acid receptors (RARs) and retinoid X receptors (RXRs), which regulate the transcription of over 500 genes. Thus, vitamin A is essential for vision and for cell differentiation and development in vertebrates including humans. Fig. 50.2 shows the structures of the major dietary retinoids and their metabolites and outlines their functions.

50.3
Dietary Sources and Forms
As mentioned above, vitamin A activity in the diet derives from two sources: preformed vitamin A as retinyl esters in foods of animal origin and provitamin A carotenoids, such as β-C, α-C, and β-cryptoxanthin, found in plant–derived foods. Stoichiometric conversion of 1 mol of β-C (with 2 β-ionone rings) gives rise to 2 mol of retinol (via retinal), whereas conversion of a mole of either β-cryptoxanthin or α-C (each with only a single β-ionone ring) gives rise to a single mole of retinol. β-C is the most potent vitamin A precursor of all provitamin A carotenoids. In order to exhibit a provitamin A activity, the carotenoid molecule must have at least one unsubstituted β-ionone ring and the correct number and position of methyl groups in the polyene chain. In practice, α-C, β-cryptoxanthin, and γ-carotene show 30%–50% of provitamin A activity and 9- cis and 13- cis isomers of β-C less than 10%, compared with all- trans β-C.
Foods in the US diet with the highest concentrations of preformed vitamin A are avian and mammalian livers, meats, instant powdered breakfast drinks, ready-to-eat cereals, and margarines. Other than liver and meats, the other sources derive their high retinyl ester contents from fortification. The highest concentrations of vitamin A as provitamin A carotenoids are found in carrots, sweet potatoes, pumpkin, kale, spinach, collards, and squash. A retinol activity equivalent (RAE) is equal to 1 μg retinol or 12 μg β-C, or 24 μg of α-C or β-cryptoxanthin. Dietary survey data shows that the major contributors to the intake of preformed vitamin A are milk, margarine, eggs, beef liver, and ready-to-eat cereals, while the major sources of provitamin A carotenoids are carrots, cantelopes, sweet potatoes, and spinach. The mean intake of vitamin A in the United States was about 600 μg RAE/day from food and 70%–75% of this was as preformed vitamin A (retinol). In contrast, most populations in the developing world get most of their vitamin A from provitamin A carotenoids in fruits and vegetables.
50.4
Solubilization of Carotenoids and Retinoids
Carotenoids are hydrophobic molecules and are thus located in lipophilic sites of the cells. To move through an aqueous environment, carotenoids can form complexes with proteins. Specific carotenoid-protein complexes have been reported mainly in plants and in invertebrates (e.g., cyanobacteria, crustaceans, silkworm). In vertebrates, data on the existence of carotenoproteins are limited. Although no intracellular β-C-binding protein was found in bovine liver and intestine, specific xanthophyll-binding proteins were reported in the human retina and macula. As an alternative mechanism for their water solubilization, carotenoids could use small cytosolic carrier vesicles. In nature, carotenoids can be also present in very fine physical dispersions (or crystalline aggregates) in aqueous media; oranges, tomatoes, and carrots are well-known examples of sources that contain such aggregates. These different physicochemical characteristics, i.e., chemical structure, positioning in biological membranes, and interaction with proteins, may account for the differences observed among individual carotenoids in their absorption and metabolism as well as their biological activities.
Retinoids are also lipophilic molecules that require solubilization. Unesterified retinol exerts detergent-like properties on cellular membranes and is usually sequestered in cells by a variety of retinol-binding proteins which are discussed in more detail below. The very nonpolar retinyl esters are usually found in cells in stabilized lipid droplets and emulsions. The digestion of retinyl esters and the conversion of carotenoids to retinoids require catalysis by enzymes that utilize these water-insoluble substrates. In some cases, the enzymes that metabolize retinoids or carotenoids are themselves hydrophobic and membrane bound.
There is little detailed information on the physical forms or “phases” that retinyl esters and carotenoids adopt in the intestinal lumen. Much more detailed information on these issues is available for other major dietary lipids such as triglycerides, phospholipids, and cholesterol. In those cases, the major phases found in the intestinal lumen are micoemulsions, micelles, mixed micelles, and unilamellar liquid crystalline vesicles. Nonetheless, it is clear from studies both in experimental animals and humans that the co-ingestion of dietary fat markedly enhances the intestinal absorption of dietary vitamin A and carotenoids. The presence of dietary fat in the intestine can stimulate retinyl ester digestion and provitamin A conversion by (1) stimulating pancreatic enzyme secretion, (2) stimulating the secretion of bile salts, which serve to form mixed micelles of lipids, and (3) providing products of lipid digestion (i.e., lysophospholipids, monoglycerides, and free fatty acids), which themselves can serves as components of micelles. Finally, fat ingestion promotes vitamin A and carotenoid absorption by providing the lipid components for intestinal chylomicron assembly, a process discussed in more detail below.
50.5
Digestion of Retinyl Esters
Almost five decades ago Erlanson and Borgström reported the partial separation of two different pancreatic retinyl ester hydrolase (REH) activities in the rat. These are now recognized to be carboxylester lipase (CEL) and pancreatic triglyceride lipase (PTL) .
Pancreatic carboxylester lipase catalyzes the hydrolysis of cholesteryl esters, triglycerides, and lysophospholipids. It was thought to hydrolyze retinyl esters also in the intestine. CEL knockout (CEL KO) mice were generated to study the functions of CEL. Although neither CEL KO nor wild-type (WT) mice absorbed non-hydrolyzable cholesteryl ether, CEL KO mice absorbed about half the amount of cholesterol provided as cholesteryl ester compared with WT mice. These data indicated that hydrolysis of cholesteryl esters is necessary prior to absorption, and that CEL plays an important role in cholesterol absorption. In contrast to the results for cholesteryl ester, CEL KO mice absorbed the same amount of retinol, when provided as retinyl ester, as did WT mice. On the other hand, neither mouse absorbed the non-hydrolyzable retinyl hexadecyl ether. These data suggested that retinyl ester hydrolysis was required for absorption and that CEL was not the responsible enzyme. The triglyceride absorption was also comparable between CEL KO and WT mice indicating that the absence of CEL does not affect triglyceride hydrolysis. Therefore, if intestinal retinyl ester absorption is unaffected in CEL KO mice, one or more other retinyl ester hydrolytic enzymes must be present in the gut lumen or on the enterocyte surface.
Studies were then conducted to identify the non-CEL pancreatic REH activity that appeared to be present in CEL KO mice, as well as to investigate this activity in WT mice and in rats. Several lines of evidence suggest that this activity is due to PTL. Although these data strongly suggest that PTL is a major REH activity in rat and mouse intestinal lumen, other enzymes synthesized and secreted by pancreas probably play a role in the lumenal hydrolysis of retinyl esters. For example, studies have suggested that pancreatic lipase-related protein 2 (PLRP2) may play a role. PLRP2 is 65% identical to PTL and hydrolyzes phospholipids and shows activity toward triglycerides in the classical PTL assay. It has been demonstrated that purified PLRP-2 (but not the related PLRP-1) can catalyze the hydrolysis of retinyl palmitate in vitro, but the activity was about one-third that of PTL. Thus, more than one enzyme may be responsible for the complete hydrolysis of retinyl esters in the intestinal lumen.
In addition to pancreatic REH activities, an REH activity intrinsically located in the brush border membrane of the absorptive enterocytes was reported in rat and human intestines. This enzyme activity was due to an intestinal phospholipase B (PLB). It is likely that PTL, PLRP2, and PLB all contribute to retinyl ester digestion.
50.6
Digestion of Carotenoid Esters
The major provitamin A carotenoid, β-C, is a hydrocarbon carotene, and thus occurs free in the diet. Consequently, it does not require enzymatic digestion prior to intestinal absorption. However, the dietary xanthophylls (viz. β-cryptoxanthin, LUT, and zeaxanthin, ZEA) are found in foods in unesterified form and as fatty acyl monoesters, and in the cases of LUT and ZEA, as diesters. The relative amounts of xanthophyll esters versus free xanthophylls differ among various fruits and vegetables.
A few studies have suggested that the intestinal absorption of xanthophylls is increased in subjects fed xanthophyll esters rather than free xanthophylls, while others have shown equivalent bioavailability of both forms when fed to humans. Moreover, only free xanthophylls were present in the plasma of individuals after feeding of either dietary form. Thus, it appears that xanthophyll esters, when present in the diet, are largely hydrolyzed prior to intestinal absorption. There is evidence from experiments using an in vitro digestion/Caco 2 cell uptake model, that pancreatic CEL is likely to be the major lipase involved in the hydrolysis of dietary xanthophyll esters (as it is for cholesterol esters cited above).
50.7
Intestinal Absorption of Provitamin A Carotenoids
50.7.1
Human Studies
Because people in developing countries primarily rely on provitamin A-containing fruits and vegetables for their vitamin A intake, the digestion and absorption of provitamin carotenoids has been well studied. A recent review by Kopec et al. provides a more thorough investigation on this topic, and discusses the uptake of all carotenoids, while herein we focus on provitamin A carotenoids. The most important factor impacting carotenoid uptake is co-consumption of lipid, with greater quantities of lipid resulting in greater absorption of provitamin A carotenoids. As previously discussed, lipid stimulates pancreatic enzyme and bile salt release into the intestine, facilitating the formation of mixed micelles. In most instances, the incorporation of carotenoids into mixed micelles (termed bioaccessibility ) very highly predicts the amount of carotenoid that is taken up by the enterocyte, packaged into chylomicrons, and ultimately released into the blood stream (termed bioavailability ). However, not all lipids are created equal. Early work by Borel et al. investigated the quantity of β-C (120 mg) absorbed 6 h postprandially after co-consumption with either 40 g medium-chain fatty acids (primarily 8:0 and 10:0) or 40 g long-chain fatty acids (primarily 18:1 and 18:2). A staggering 4.42-fold increase in total β-C was absorbed when consumed with the long-chain fatty acids as compared to the medium-chain fatty acids. Recent work by Goltz et al. investigated the effects of differing doses of butter (rich in medium-chain saturated fatty acids), soybean (polyunsaturated long-chain fatty acids), and canola (monounsaturated long-chain fatty acids) oils. A nonsignificant trend toward greater absorption of α-C and β-C was observed when consumed with 3 and 8 g canola oil as compared to butter, although this trend did not hold when consumed with 20 g lipid. It is possible the complex study design was underpowered to observe statistical differences.
The capacity of lipid to increase provitamin A absorption likely has an upper maximum, relative to the dose of provitamin A fed, as well as the dose of lipid fed. A crossover study in healthy humans ( n = 11) showed no difference between the amount of α- and β-C absorbed from a mixed salad, irrespective of whether the salad was co-consumed with avocado delivering 12 g of lipid, avocado delivering 24 of lipid, or 24 g of purified avocado oil. It should be noted that the profile of avocado lipid is almost identical to that of canola oil. With regard to β-C dose, a doubling of d8 β-C dose (from 20 to 40 mg) produced a doubling of 15 h postprandial AUC in the seven subjects tested. It is not clear at which dose the percent of β-C absorption is reduced, but a maximum likely exists.
Food matrix also has a large impact on carotenoid bioavailability. Thermally processed vegetables have been shown to increase bioavailability of provitamin A carotenoids as compared to the raw or less processed counterpart. Women ( n = 8) in a crossover study consumed raw carrots + spinach daily for 4 weeks followed by processed carrots + spinach for 4 weeks. Provitamin A levels (9.3 mg β-C) were dose matched between the two feeding treatments. A threefold increase in circulating plasma levels of β-C was observed after processed spinach + carrots were consumed, relative to the raw version. These results were further corroborated by work in ileostomy volunteers consuming 15 mg β-C from cooked or pureed carrot, where a 58% increase in postprandial β-C was observed with the cooked carrot relative to raw carrot. Another study investigated the impact of progressively stronger processing treatments on β-C bioavailability from carrots. Raw chopped carrot, boiled mashed carrot, and processed carrot puree dose matched to deliver 18.6 mg of β-C were fed to six individuals. As expected, the stronger conditions used to process carrot puree delivered approximately twofold greater β-C than the boiled mashed carrot. The amount of β-C absorbed from the raw carrot was less than that of the puree, although surprisingly greater than the boiled mashed carrot in four of six subjects, clearly demonstrating that high interindividuality in other factors can overwhelm processing effects.
Beyond heat treatment, food form can change the matrix and affect absorption. In a study by van het Hof et al. whole, peeled, and canned tomatoes were treated with progressively stronger levels of homogenization (none, mild, and severe). Subjects ( n = 11) consumed each level of homogenized tomato in a three-way crossover. A ninefold increase in postprandial AUC levels of β-C was observed for the severe homogenization meal as compared to the no homogenization meal. Pasteurized orange juice has also been shown to increase postprandial β-cryptoxanthin levels approximately twofold greater than an equivalent dose of β-cryptoxanthin consumed from the raw oranges, presumably due to processing and fiber differences.
Differences in the physicochemical states of carotenoid storage in plants also impact bioavailability, as recently reviewed. Although much work in this area has been done in vitro, one study investigated the bioavailability of β-C from raw papaya versus raw tomato and raw carrot. β-C from raw papaya (where the carotenoid is stored in lipid droplets) was significantly greater than the bioavailability from raw carrots and raw tomatoes (where the carotenoid is stored in solid-crystalline form) in a three-way crossover study.
Studies have demonstrated reduced bioavailability of β-C over 12 h postprandially when fed with a second carotenoid (i.e., LUT and LYC), as compared to β-C fed alone. These results would be anticipated considering the sharing of facilitated uptake transporters (SR-B1 and others, as further discussed below). However, this reduction in short-term uptake may not impact on longer-term carotenoid status. Co-consumption of phytosterols have also been shown to reduce provitamin A uptake under very low fat meal conditions, although these results have not been validated with normal or high meal lipid co-consumption.
Isotope studies of β-C have estimated that 1.2%–22% of provitamin A carotenoid is bioavailable from a variety of sources and food matrices, as summarized by van Lieshout et al. and reported by others. However, when very low doses of carotene are fed in oil, much higher bioavailability (53%–81%) is reported.
Repeated carotenoid dosing increases blood levels to a point. In a study of 64 men and women, the feeding of a daily dose of 12.4 mg combined with α- and β-C (in a lipid-rich capsule) over a 20-week time period revealed that fasting blood levels peaked at an average of 2.25 μmol/L at 8 weeks, and levels did not increase further over the remaining weeks of the study period. Studies in healthy adults (using isotope tracers) have demonstrated the half-life of β-C consumed orally to be 13–35 days in blood plasma.
50.7.2
Mechanisms
Given the limitations of using human subjects, much of our knowledge of the molecular mechanisms of intestinal carotenoid absorption has come from studies of in vitro intestinal cell culture model systems that mimic the in vivo intestinal absorption of carotenoids and other lipids. Highly differentiated Caco-2 cells cultured on membranes are able to form and secrete chylomicrons that have characteristics similar to those of chylomicrons secreted in vivo.
Based on early rat studies, the intestinal absorption of carotenoids was thought to be a passive diffusion process determined by the concentration gradient of the carotenoid across the intestinal membrane. However, the kinetics of β-C transport through Caco-2 cell monolayers suggested that the intestinal transport of carotenoids is facilitated by the participation of a specific epithelial transporter. Of the total β-C secreted by Caco-2 cells 80% was associated with chylomicrons, pointing to the importance of chylomicron assembly for β-C secretion into the lymph in vivo, as it is for retinyl ester secretion.
Human studies have consistently reported a preferential accumulation of all- trans -β-C in plasma, compared with its 9- cis isomer (which can form during processing of β-C-containing foods). When three geometrical isomers of β-C were applied separately to Caco-2 cells both 9- cis- and 13- cis -β-C were taken up by the cells to only the extent of one-fifth of all- trans -β-C. In addition, two carotenoids exhibiting similar structural characteristics competed with each other for their cellular uptake. These data are consistent with the idea of a facilitated uptake process.
The molecular mechanism of facilitated transport of carotenoids in human intestine was suggested by the identification of a scavenger receptor with a high sequence homology to the mammalian class B scavenger receptors (SR-B1 and CD36) that mediated the cellular uptake of carotenoids in Drosophila. Further work in Caco-2 cells has demonstrated a direct role for SR-B1 in the cellular uptake of β-C, LUT, and LYC. Moreover, studies of the SR-B1 knockout mouse indicate a role for this lipid transporter in the intestinal absorption of β-C in the intact animal. Interestingly, the intestinal absorption of carotenoids is controlled by RA-induced expression of the transcription factor ISX which then represses expression of SR-B1.
50.8
Conversion of Provitamin A Carotenoids to Retinoids
50.8.1
Carotene Cleavage Enzymes
After uptake of provitamin A carotenoids by human intestinal cells they are partly cleaved at the central double bond to yield one or two molecules of retinaldehyde (retinal). This conversion is catalyzed by the enzyme, β-C 15,15′ oxygenase. The retinal so produced is reduced to retinol, which can then be either absorbed as such or reesterified to long-chain retinyl esters for incorporation into chylomicrons.
Early studies on carotenoid metabolism in animals focused on provitamin A carotenoids and their enzymatic conversion to vitamin A. The first in vitro studies published in 1965 used extracts from rat liver and intestinal mucosa to demonstrate the enzymatic oxidative cleavage of β-C at the central double bond to yield retinal. In 2000, the enzyme responsible for the conversion of β-C to retinal was identified in Drosophila and chicken and was named β-C-15,15′-oxygenase 1 (BCO1) 1 . BCO1 has also been identified in humans, mice, rat, cows, zebrafish, and Caenorhabditis elegans. In 2001, another carotenoid cleavage enzyme that catalyzes eccentric cleavage, β-C-9′,10′-oxygenase (BCO2) was identified in human, mouse, and zebrafish. Fig. 50.3 shows the cleavages of β-C catalyzed by BCO1, BCO2, and as yet undefined mechanisms.

More recent work on the enzymology of vertebrate BCO1 and BCO2 has clarified the substrate specificity, kinetics, and mechanisms of these two carotenoid oxygenases. In this work, the substrate specificity of purified recombinant human BCO1 was assessed in terms of catalytic efficiency values (kcat/KM). The purified enzyme preparation catalyzed the oxidative cleavage of β-C with a Vmax = 197.2 nmol retinal/mg BCO1 × h, KM = 17.2 μM and catalytic efficiency kcat/KM = 6098 M − 1 min − 1 . The enzyme also catalyzed the oxidative cleavage of α-C, β-cryptoxanthin and β-apo-8′-carotenal, β-apo-10′-carotenal, β-apo-12′-carotenal, and β-apo-14′-carotenal to yield retinal directly. The catalytic efficiency values of these substrates are lower than that of β-C. This lower catalytic efficiency with the latter substrates is consistent with their lower provitamin A activity when provided in the diet. Surprisingly, BCO1 catalyzed the oxidative cleavage of LYC to yield acycloretinal with a catalytic efficiency similar to that of β-C. The enzyme did not catalyze the cleavage of LUT, ZEA, and 9- cis β-C. Thus, BCO1 favors full-length provitamin A carotenoids as substrates, with the notable exception of LYC. A subsequent study of this enzyme established that its reaction mechanism was a dioxygenase and not a monooxygenase as had been suggested previously.
Similar studies were conducted with purified recombinant BCO2. Like BCO1, purified recombinant chicken BCO2 reacts with the provitamin A carotenoids β-C, α-C, and β-cryptoxanthin. Its catalytic activity with β-C as substrate is 10-fold less than that of BCO1. In further contrast to BCO1, purified recombinant chicken BCO2 also reacts with 9- cis -β-C and non-provitamin A carotenoids ZEA and LUT, and does not react with all- trans -LYC and β-apocarotenoids. Apo-10′-carotenoids were detected as enzymatic products by high-performance liquid chromatography (HPLC). Thus, BCO2 cleaves specifically at the 9′-10′ bond to produce apo-10′-carotenoids. The different substrate specificities of BCO1 and BCO2 are illustrated in Fig. 50.4 .

The contribution of BCO1 and BCO2 to the production of vitamin A was established by careful study of BCO1 and BCO2 KO mice. BCO1 −/−, BCO2 −/−, or BCO1 −/−/BCO2 −/− double KO mice were fed a diet containing β-C as the sole source of vitamin A. Genetic disruption of BCO1 resulted in vitamin A deficiency, which demonstrated the critical role of the enzyme in retinoid production. Disruption of BCO1 was accompanied by a BCO2-dependent production of small amounts of β-apo-10′-carotenol which was then converted to vitamin A by BCO1.
The two major sites of β-C conversion to vitamin A in humans are the intestine and liver. By direct determination of BCO1 activity in human small intestine and liver samples, it was estimated that in a human adult the maximum capacity for β-C cleavage by the two organs combined was 12 mg β-C/day. This amount is much higher than the observed average daily intake of 1.5 mg β-C/day in the United States or even the higher daily intake of 6 mg β-C/day suggested by some authors as being needed to meet the goal of 90% of vitamin A intake. Human liver was shown to have four times greater capacity for metabolizing β-C than the small intestine. This is consistent with the earlier prediction, using a multicompartmental model, that in humans β-C cleavage takes place in liver to a greater extent than in intestine. In rats, highest activities of BCO1 were also found in small intestine and liver followed by brain, lung, and kidney. In agreement with the tissue distribution of the BCO1 activity, high levels of human BCO1 mRNA were reported in the jejunum, liver, and kidney, whereas lower levels were present in the prostate, testis, ovary, and skeletal muscle. In contrast to humans, rats convert most of β-C in intestine and only small amounts of β-C reach the liver
In rats, BCO1 activity was enhanced by vitamin A deficiency, dietary polyunsaturated fats, copper depletion, and fructose feeding and inhibited by protein deficiency. A direct repeat of RA response elements (RAREs) was identified in the promoter of the mouse BCO1 gene, suggesting that RA and 9- cis RA could downregulate the intestinal enzyme in rat and chicken at the transcriptional level via their interaction with RARs and/or RXRs. This mechanism of regulation could be generalized to all pro-RA retinoids and carotenoids and explains why vitamin A deficiency status enhanced the intestinal enzyme activity. As with the regulation of SR-B1, the mechanism involves the RA-dependent induction of the intestinal transcription factor, ISX, and the ISX-induced repression of BCO1 expression. The details of this mechanism have been defined. The ISX binds to the BCO1 promoter. After treatment with RA, this ISX binding decreased expression of a luciferase reporter gene in human colonic Caco-2 cells indicating that ISX acts as a transcriptional repressor of BCO1. Mice deficient for this transcription factor displayed increased intestinal BCO1 expression and produced significantly higher amounts of vitamin A from supplemental β-C. Importantly, the ISX binding site in the human BCO1 promoter contains a common single nucleotide polymorphism (SNP) that is associated with decreased efficiency of β-C conversion to vitamin A and increased plasma levels of β-C. The molecular and dietary regulation of BCO1 has been the subject of two excellent reviews.
50.9
Intestinal Absorption of Vitamin A
50.9.1
Human Studies
Human studies often feed vitamin A in the form of retinyl acetate or retinyl palmitate, the two most common forms of vitamin A also used for the supplementation of dairy and fortified cereals. Approximately, 50%–90% of preformed vitamin A has been shown to be absorbed when physiologically relevant doses are provided, and has been recently reviewed.
A portion of provitamin A is converted in the intestine to retinal, which is then reduced to retinol before esterification to produce retinyl esters. When greater doses of provitamin A are fed, a lower percentage is converted into vitamin A. The administration of a 40 mg dose of labeled β-C resulted in a doubling of β-C, but only a 36% increase in labeled plasma retinol, relative to a 20 mg dose in the same subject cohort. Thus, the body appears to regulate conversion of provitamin A to vitamin A, likely protecting the organism from the effects of vitamin A toxicity. Indeed, individuals who overconsume provitamin A carotenoids develop an orange-like hue to their skin, termed carotenemia. This benign condition can be differentiated from jaundice due to the absence of a yellow hue to the conjunctiva. The symptoms associated with preformed vitamin A toxicity include teratogenesis in developing fetuses, as well as adverse skin reactions, weakening of the bones, and ultimately liver cirrhosis.
It should also be noted that intestinally converted provitamin A is preferentially esterified to palmitic acid, and retinyl palmitate comprises approximately 75% of the retinyl esters found in chylomicrons. The percentage of β-C which is immediately converted in the intestine to vitamin A can be quite high, especially when large doses are fed. The contribution of asymmetric provitamin A carotenoids to the vitamin A pool has also been a topic of recent interest, with new methods developed for better assessment of conversion efficiencies of α-C. In a human study feeding with carrots, it was predicted that α-C would contribute 25% of the newly formed retinyl esters based on relative dose, but a significant amount of interindividual variability (12%–35%) was observed.
Vitamin A status in humans is difficult to ascertain without isotopes, as decreases in serum retinol levels (< 0.7 μmol/L) only occur shortly before severe symptoms of deficiency (xerophthalmia, issues of dark adaptation) are observed. Levels of 0.7–1.05 μmol/L are considered marginal . For an accurate assessment of total body vitamin A stores (most especially liver stores), an isotope dilution method has been traditionally used which was first tested in rats before it was assessed in humans as a single and double dilution method. Continued improvements in isotope measurement and compartmental modeling have been made in recent years [for a review see Lietz et al. ].
Lipid is important for the uptake of retinol. Long-chain fatty acids produce a significantly increased postprandial retinyl ester response compared with medium-chain fatty acids when retinyl palmitate is fed. Lipid also appears to facilitate conversion of provitamin A to vitamin A. A crossover study was in healthy subjects fed a β-C rich tomato sauce meal with and without avocado (delivering 23 mg lipid). Retinyl esters were measured 12-h postprandially, and a 4.6-fold greater increase in AUC retinyl esters was observed. Notably, a greater than anticipated conversion of provitamin A to vitamin A was observed in subjects who were less efficient provitamin A converters when the meal was fed without avocado. In other words, less efficient converters produced more vitamin A than would have been predicted simply from the increased uptake in provitamin A when avocado was co-consumed.
50.9.2
Mechanisms of Cellular Uptake and Efflux of Unesterified Retinol in Enterocytes—Portal Secretion
Studies concerning the uptake of retinol by the human intestinal cell line, Caco-2, indicated that under postprandial conditions (i.e., given oleic acid) retinol taken up by these cells was esterified and the retinyl esters were secreted with chylomicrons. In contrast, under “fasting” conditions the cells do not secrete retinyl esters but secrete retinol not associated with lipoproteins into the basolateral medium.
Other experiments have further suggested the role transporters in retinol flux in Caco-2 cells. When cells were incubated with retinol for varying times up to 24 h, cellular retinol plateaued within 2 h, whereas there was continuous formation of retinyl esters. Both retinol and retinyl esters secreted in basolateral medium increased linearly with time (up to 20 h). Retinyl esters were associated with chylomicrons and retinol with the nonlipoprotein fraction. Cellular uptake of retinol was directly proportional to initial retinol concentration. However, the kinetics of efflux of retinol into basolateral medium revealed two processes. Retinol secretion showed saturation at concentrations < 10 μM, implying a mediated transport out of the cell, and linearity with higher concentrations, implying passive diffusion. One interpretation of these data is that free retinol enters into intestinal cells by simple diffusion, while its secretion may require a facilitated transport at physiological doses. Glyburide, a known inhibitor of the ABCA1 transporter, caused marked inhibition of the efflux of free retinol into basolateral medium (but not cell uptake). Finally, inhibition of ABCA1 protein expression by siRNAs decreased retinol efflux in proportion to the extent of protein knockdown. Early studies using intestinal segments also suggested that the unesterified retinol was absorbed by protein-mediated facilitated diffusion and passive diffusion mechanisms at physiologic and pharmacological concentrations, respectively.
Thus, both carotenoids and retinol join a growing list of fat-soluble molecules that are absorbed via the action of membrane-bound lipid transporters. For example, three different membrane-bound proteins, CD36, membrane-bound fatty acid-binding protein, and a fatty acid transport protein, that are involved in fatty acid uptake have been identified [for a review, see Abumrad et al. ; Glatz et al. ]. In the case of cholesterol, SRB1, CD36, NPC1L1, and a variety of ABC transporters have been implicated in its uptake and/or efflux from various cells . It is possible that these or other proteins may play a role in the transport of retinol across cell membranes. Defining the exact mechanisms of cellular uptake of retinol (or other lipids) is complicated by the fact that, as indicated above, multiple mechanisms (both facilitated and passive) may exist in a single cell. An additional problem is that much of the work in this area relies on the use of membrane transporter inhibitors, and there is increasing evidence that some of these compounds inhibit multiple transporters types. A final complication is that some of the transport inhibitors also affect the expression of transporter genes and, thus, exert effects on transporter number and function simultaneously.
The general perception that retinol is efficiently absorbed and quantitatively transported on chylomicrons may need reevaluation [for a review see Blomhoff et al. ]. First, it should be pointed out that the recovery of ingested retinol into lymph varies between 20% and 60% in various studies. Second, Hollander showed that approximately 60% and 30% of the absorbed retinol is secreted into lymph and portal circulation, respectively. Third, oral supplementation of retinol in abetalipoproteinemia patients, who do not assemble and secrete chylomicrons, results in partial recovery from symptoms of retinol deficiency. Fourth, cell culture studies showed that free retinol or its metabolized products are transported across the cells independent of the assembly and secretion of lipoproteins. Thus, much of the absorbed retinol is secreted into lymph in esterified form. However, a significant amount is also secreted into portal circulation probably as free retinol. The transport of free retinol to the portal circulation is expected to be physiologically significant in pathologic conditions that affect the secretion of chylomicrons. Thus, the transport of free retinol may be an essential backup mechanism for the homoeostasis of vitamin A under some conditions.
50.9.3
Intracellular Transport by CRBP(II)
After cellular uptake, free retinol is probably immediately sequestered by cellular retinol binding proteins (CRBPs). Two CRBPs, CRBP(I) and CRBP(II), have been purified and characterized extensively. They have considerable sequence identity and belong to a family of lipid-binding proteins (the lipocalins). Both the proteins are highly conserved during evolution indicating their physiologic importance. These proteins share considerable structural, genetic, and biochemical properties. However, the cellular expression pattern of these proteins is very different. The CRBP, a 14.6 kDa polypeptide, is expressed in many tissues whereas CRBP(II), a 16 kDa polypeptide, is expressed primarily in the absorptive cells of the small intestine. The CRBP(II) is one of the most abundant proteins and accounts for approximately 1% of the total soluble proteins recovered from the jejunal mucosa. The tissue distribution and abundance indicate that it must be uniquely suited for retinol absorption by the intestine [for reviews see Li and Norris ; Newcomer et al. ; Ong ].
In vitro studies indicated that CRBP(II) can play several functions in the trafficking of retinol. It has been speculated that it can bind to specific transporters on the brush border membrane and permit facilitated diffusion. It can serve as a reservoir to keep the concentrations of free retinoids very low and protect cells from their detergent-like properties. More important, it may present retinoids to different enzymes and direct their metabolism.
In vivo studies showed that CRBP(II) mRNA levels increased in the small intestine of the retinoid-deficient rats, and rats fed with long-chain fatty acids. In Caco-2 cells, CRBP(II) mRNA was shown to be increased (two- to threefold) after treatment of the differentiated cells with RA More importantly, this resulted in increased absorption and intracellular esterification of radiolabeled retinol. Furthermore, absorption and esterification also increased after the overexpression of CRBP(II) in these cells. These studies indicate that changes in CRBP(II) expression results in the modulation of retinol metabolism. However, it is not known whether the increased expression of CRBPII results in increased secretion of retinyl esters in chylomicrons.
The characterization of the CRBPII knockout mouse has clarified that CRBPII plays an important, but not absolutely essential, role in the intestinal absorption of vitamin A. Thus, the saturable component of retinol uptake by jejunal segments was reduced to about half that of tissue from WT littermates. The CRBPII KO mice, when maintained on a vitamin A-enriched diet, also had reduced (about 40%) hepatic stores of vitamin A, suggesting that the incomplete impairment of retinol processing in the intestine nonetheless affects whole body vitamin A status.
50.9.4
Reesterification and Incorporation Into Chylomicrons—Lymphatic Secretion
Early studies in intact rats and humans clearly demonstrated that after uptake of newly absorbed retinol, the retinol was largely reesterified with long-chain fatty acids (mostly palmitate) and secreted into the lymphactics along with other dietary lipids in chylomicrons. In enterocytes, two enzymes, lecithin:retinol acyl transferase (LRAT) and acyl-coA:retinol acyltransferase (ARAT), have been identified that can catalyze the esterification of free retinol in vivo. It has been suggested, but not shown, that retinyl esters formed by LRAT and ARAT may be targeted for secretion with chylomicrons and storage, respectively. The characterization of the LRAT KO mouse has clearly demonstrated that LRAT plays the major physiological role in retinol esterification. However, it is also clear that diacylglycerol acyltransferase 1 (DGAT1) acts as an intestinal ARAT involved in the esterification of retinol especially when pharmacologic doses of retinol are given to animals.
It is generally believed that retinol is mainly secreted into the lymph as retinyl palmitate. During metabolic studies, analysis of the plasma revealed that most of the retinyl esters are present in small chylomicrons. Significant amounts of retinyl esters are also found in large chylomicrons followed by smaller amounts in very low-density lipoproteins. In contrast to triglycerides, cholesterol esters, and other lipids, retinyl esters are not present in other lipoproteins such as intermediate density lipoproteins, low-density lipoproteins, or high-density lipoproteins. These studies indicate that retinyl esters are mainly present in large and small chylomicrons and behave very differently from other neutral lipids, such as triglycerides and cholesterol esters.
To understand the mechanism of secretion of RE by the intestine under the fasting and postprandial states, studies were conducted where differentiated Caco-2 cells were supplemented with radiolabeled retinol under conditions that support (postprandial) or do not support (fasting) chylomicron secretion. These cells assimilated vitamin A by a very rapid uptake mechanism under both conditions. After uptake, the cells stored retinol in both esterified and unesterified forms. Under fasting conditions, the cells mainly secreted variable amounts of free retinol unassociated with lipoproteins. However, under postprandial conditions, these cells secreted significant amounts of retinyl esters mainly with chylomicrons. The secretion of RE with chylomicrons was independent of the rate of uptake of retinol, intracellular free, and esterified retinol levels, and was dependent on the assembly and secretion of chylomicrons. Inhibition of chylomicron secretion by Pluronic L81 decreased the secretion of RE and did not result in their increased secretion with smaller lipoproteins. These data strongly suggest that RE secretion by the intestinal cells is a highly specific and regulated process that is dependent on the assembly and secretion of chylomicrons. Retinyl ester secretion does not occur at all times. It is induced when cells can assemble and secrete chylomicrons. Thus, it appears that intestinal cells may have a specific mechanism for the targeting of retinyl esters to nascent chylomicrons.
As discussed above, Caco-2 cells do not secrete retinyl esters under conditions simulating the fasting state. In the work on the kinetics of retinol uptake by Caco2 cells, a 5-day “washout” experiment was conducted in which cells were incubated with retinol for 16 h to accumulate cellular retinol and retinyl esters and then incubated with retinoid-free medium (containing fatty acids and thus mimicking the “fed” state) that was changed every 24 h. This resulted in the release of the free retinol, but not the accumulated retinyl esters. This implies that only newly synthesized retinyl esters are incorporated into chylomicrons and that preformed retinyl esters cannot be used for CM assembly. Thus, the synthesis of retinyl esters and their incorporation into chylomicrons appear to be a concerted processes.
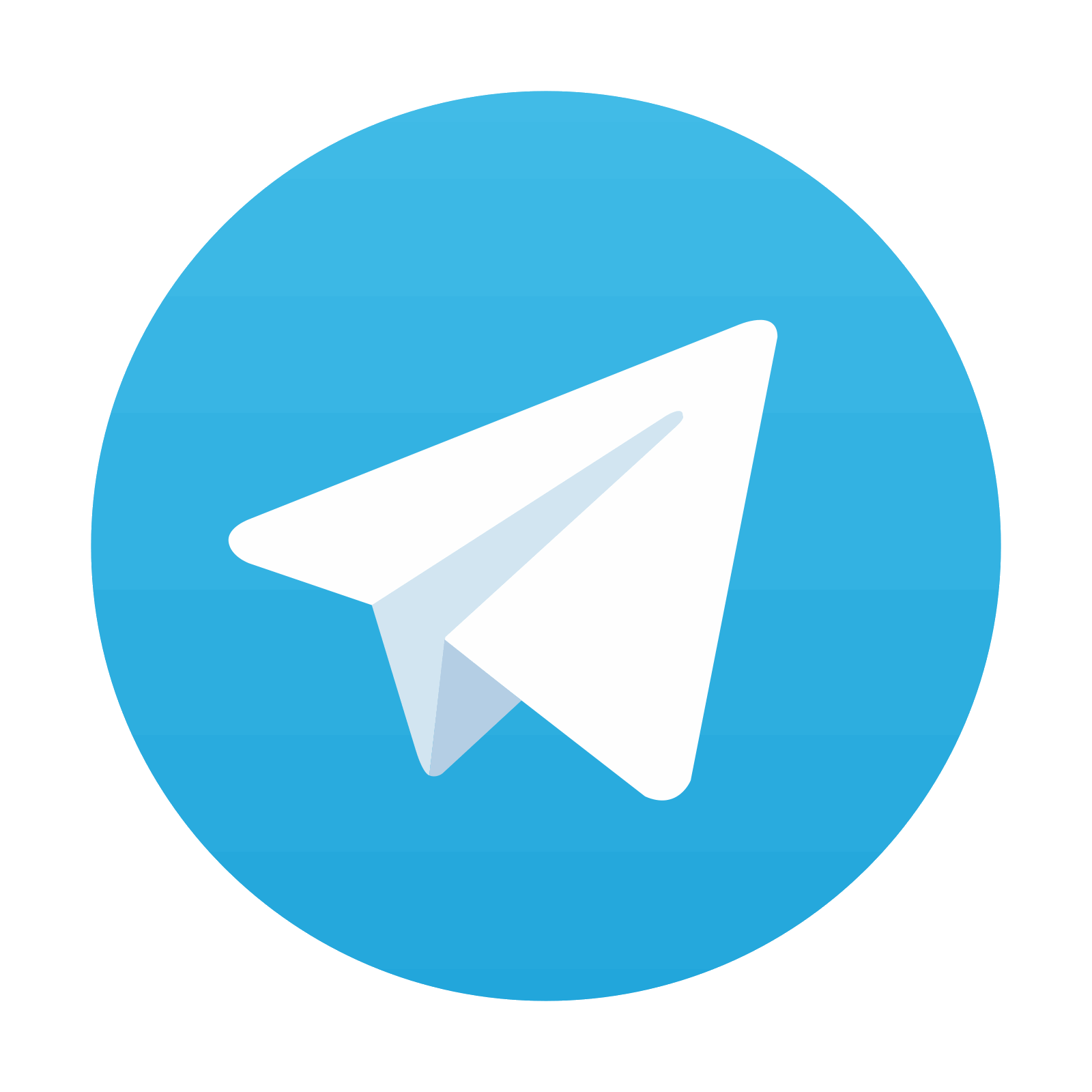
Stay updated, free articles. Join our Telegram channel
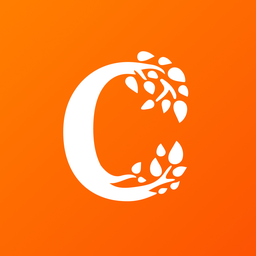
Full access? Get Clinical Tree
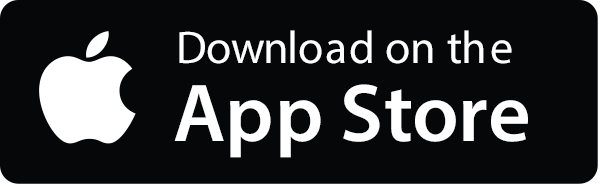
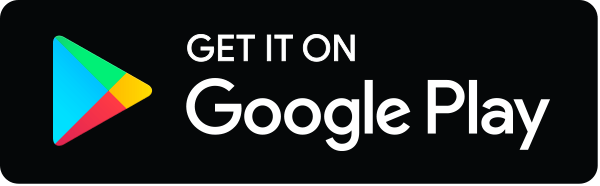