The treatment of prostate cancer with low dose rate prostate brachytherapy has grown rapidly in the last 20 years. Outcome analyses performed in this period have enriched understanding of this modality. This article focuses on the development of a real-time ultrasound-guided implant technique, the importance of radiation dose, trimodality treatment of high-risk disease, long-term treatment outcomes, and treatment-associated morbidity.
The treatment of prostate cancer with low-dose-rate prostate brachytherapy has grown rapidly over the last 20 years. Outcome analyses performed in this period have enriched understanding of this modality. Many topics can be covered in a review of this subject, but limitations of the current article format prevent a thorough comprehensive review of all of these. Instead, this article focuses on a more limited number of topics that the authors believe are relevant to current understanding of brachytherapy. These topics include the development of a real-time ultrasound-guided implant technique, the importance of radiation dose, trimodality treatment of high-risk disease, long-term treatment outcomes, and treatment-associated morbidity.
The development of a real-time ultrasound-guided implant technique
The advent of transrectal ultrasound-guided brachytherapy was first reported by Holm and Gammelgaard in Denmark in 1981. These investigators described the use of transrectal ultrasound to help guide needle and seed placement in the treatment of localized prostate cancer using iodine 125 ( 125 I) radioactive seeds. In the last 26 years, there have been many significant advances in low-dose-rate brachytherapy techniques, all derived from this initial approach. This article outlines the real-time method of ultrasound-guided implantation developed at Mount Sinai Hospital, New York, in 1990.
The original technique was based on the concept that a preplan of seed placement could never be exactly duplicated in the operating room because of changes in the shape of the prostate and its position relative to the bladder and rectum. Instead, a technique was developed to take into account prostate mobility and use the imaging capabilities of transrectal real-time ultrasound. Using ultrasound, the physician could monitor the position of the prostate during the operation and the seeds could be placed based on the live image of the gland. To further simplify the planning aspect, a lookup table was developed. The amount of radioactivity to implant was derived from the original Memorial Sloan Kettering Cancer Center (MSKCC) nomogram for 125 I volume implants. This nomogram allowed calculation of the amount of radioactivity to implant based on the volume measured to deliver a matched peripheral dose of 160 Gy.
The basic concept behind the technique is simple and consists of 2 phases. The first phase is called the peripheral phase and involves insertion of needles into the largest transverse diameter of the gland approximately 1 cm apart. Approximately 75% of the radioactivity that is implanted is placed within the prostate via these needles. The seeds are distributed evenly throughout these needles, although needles traversing longer lengths of prostate tend to deposit 1 or 2 more seeds than shorter-length needles. The exact position of the needles and seed deposition is monitored by longitudinal live ultrasound imaging. The second phase involves the placement of interior needles (usually 5–8 needles) in such a way that the needles cover the apex and base of the gland. The remaining 25% of the activity is implanted through these needles. In the original technique, usually 3 to 4 seeds were deposited per needle. The exact placement of seeds via these needles is also monitored with longitudinal ultrasound imaging.
The final version of the Mount Sinai Hospital lookup table for activity per volume to be implanted was developed by using a quality insurance program that was based on monthly examination and review of the computed tomography (CT)-based postimplant dosimetric analyses of all implants. This quality improvement program led to an overall increase in the activity implanted per volume compared with the original MSKCC nomogram. In addition, improvements in ultrasound technology led to better visualization and more accurate placement of sources. The final amount of activity implanted per volume used in the Mount Sinai lookup table was found, in a paper by Bice and colleagues, to be consistent with other preplanning implant centers.
The next development in the technique of real-time ultrasound-guided seed implantation was the implementation of an intraoperative computer planning system. This system allowed for real-time capture of ultrasound prostate images. These prostate images could be captured with needles in place. This feature enabled the physician to recreate the actual implant in 3 dimensions on the computer. The program then allowed for interactive monitoring of delivered dose based on needle and seed deposition positions. In addition, it allowed for fine-tuning of needle positions and overall activity implanted. In a study by Stone and colleagues intraoperative ultrasound dosimetry findings were compared with 1-month CT-based postimplant results. Although small differences existed between the intraoperative and CT dosimetry results, these data suggested that this intraoperative implant dosimetric representation system provided a close match to the actual delivered doses. It supported the concept that intraoperative dosimetry could be used to modify the implant during surgery to achieve more consistent dosimetry results.
Brachytherapy and radiation dose
Radiation for prostate cancer is usually described by its physical prescription dose. For example, a patient receiving high-dose external beam radiotherapy (EBRT) for prostate cancer might be prescribed 81 Gy, which is typically delivered in 1.8-Gy fractions per day for 45 days. For a patient being treated with a permanent implant with 125 I, the typical prescription dose is 145 to 160 Gy and for those receiving palladium-103 ( 103 Pd), 125 to 130 Gy. Patients with high-risk disease are often offered a combination of external beam irradiation and seed implantation. For these patients the dose of EBRT and the implant are reduced, usually to 45 Gy (1.8 Gy ×25) and 110 Gy for 125 I or 100 Gy for 103 Pd, respectively. One of the differences between EBRT and seed implantation is the way the dose is delivered. For EBRT, frequent evaluations of treatment setup can be performed by image guidance with fiducial markers or cone beam CT to help ensure correct prostate position and treatment accuracy. On the other hand, permanent implantation is a single treatment and the delivered dose (implant quality) is determined by the posttreatment dosimetry study. This study is performed within 30 days of the implant using CT imaging and specialized software that allows calculation of the radiation dose to the prostate, rectum, urethra, and any other contiguous structures of interest. Because the modern transperineal ultrasound-guided seed implant is new, studies that report oncologic outcomes based on postimplant dose data are critically important to evaluate the efficacy and safety of the treatment.
The dose to the prostate can be described by several different parameters, for example the dose to 100%, 95%, or 90% of the prostate volume. These doses are typically termed the D100, D95, or D90. The volume of the prostate covered by the prescription dose has also been described and is designated by a “V” followed by the percentage of the dose prescribed. For example, V100 refers to the volume of the prostate covered by 100% of the prescription and V150 represents the volume covered by 150% of the prescription. The same dose parameters are also used for the critical structures. The V100 of the rectal wall represents the volume of the rectum (in cubic centimeters) covered by 100% of the prescription dose. Although not all agree, the 2 most representative descriptives of the prostate are the D90 and V150 and for the rectum the V100. In general, a higher D90 is preferred but without increasing the V150 above 50%–75%. Because the dose deposition is highest next to the radioactive source and decreases exponentially farther away from it, the implant tends to be hotter in the middle of the gland (where the urethra is) because it is surrounded by seeds. The ideal implant technique distributes these hot nonhomogeneous regions away from the urethra and keeps the V150 below 50%–75%.
Stock was the first to describe the use of the D90 to report biochemical outcomes and dose response following 125 I prostate brachytherapy. Patients receiving a D90 less than 140 Gy had a 4-year freedom from biochemical failure (FBF) rate of 68% compared with a rate of 92% for those receiving a D90 of 140 Gy or greater ( P = .02). Several other investigators have reported similar findings. Miles and colleagues investigated 145 patients and separated them by dose. The biochemical disease-free rate for patients with a D90 greater than 140 Gy was 100% compared with 90% for those with the lower dose ( P = 0.02). Recently, Kao reported on the potential for higher doses to improve results further. A total of 643 patients were treated with 125 I monotherapy for T1 or T2 prostate cancer with a D90 of 180 Gy or greater (median, 197 Gy; range, 180–267 Gy). Using the Phoenix definition, 5-year biochemical disease-free survival rates were 97.3% for low-risk patients and 92.8% for intermediate-/high-risk patients.
Unlike the prescription dose, which represents the physical dose given to the target organ, the biologic effective dose (BED) is a measure of how the dose affects the target tissues. It also permits the calculation of a common dose (in Gy) regardless of the method or rate of the delivered radiation dose. The equation for calculating the BED for the implant is: BED = (R 0 /λ){1+[R 0 /(μ+λ)(α/β)]}, where R 0 is the initial dose rate of implant = (D90)(λ), λ is the radioactive decay constant = 0.693/T 1/2 , T 1/2 is the radioactive half-life of the isotope, μ is the repair rate constant = 0.693/t 1/2 , and t 1/2 is the tissue repair half-time. For EBRT the BED = nd [1 + (d/α/β)], where n is the number of fractions given, d is the dose per fraction, and α/β is the tissue- and effect-specific parameter associated with the linear-quadratic model. The BED for the implant is highly dependent on the D90, or the dose delivered to 90% of the gland based on the postimplant dosimetry. For patients receiving EBRT and implantation, the BED is the sum of the BED calculated for the implant and the EBRT component. When the BED is used to report dose data, the source of radiation becomes less important because all of the physical prescription doses are reduced to 1 common variable, simplifying data analysis.
Stock and colleagues used the BED to report the biochemical and local control rates following prostate brachytherapy. They found increasing biochemical freedom from failure (BFFF) rates as the BED increased from 100 Gy to 200 Gy. The 10-year freedom from prostate-specific antigen (PSA) failure for the BED groups (<100, >100–120, >120–140, >140–160, >160–180, >180–200, and >200) were 46%, 68%, 81%, 85.5%, 90%, 90%, and 92%, respectively ( P <.0001). Recently, Taira and colleagues reported similar findings, in which patients with higher BEDs had improved biochemical control. Patients with a BED more than 116 Gy had a 98.8% BFFF rate compared with 92.1% for lower doses.
The ideal treatment of prostate cancer should be the one that offers the greatest chance of local disease eradication. Historically, radiation therapy (RT) has been believed to be inferior to prostatectomy because the delivered dose was not high enough to eradicate all of the tumor. However, recent advances in prostate imaging have increased the precision of prostate brachytherapy. This development should result in a dose-response relationship for local control. Stone and colleagues explored this issue by performing multicore prostate biopsies 2 years after brachytherapy in 584 men. The median PSA concentration was 7.1 ng/mL and the median follow-up was 7.1 years. There were 260 (44.5%) low-risk, 141 (24.1%) intermediate-risk, and 183 (31.4%) high-risk patients. The median BED was 186 Gy 2 (25%–75%, 158–208 Gy). Of 548 patients, 48 (8.2%) had a positive biopsy specimen at the last follow-up. The number of patients with positive biopsy results by BED group was as follows: 22 of 121 (18.2%) for patients who received less than 150 Gy 2 ; 15 of 244 (6.1%) for patients who received more than 150 to 200 Gy 2 ; and 6 of 193 (3.1%) for patients who received more than 200 Gy 2 ( P <.001). These dose groups remained significant even when patients were divided into risk group. Thus, even for low-risk patients, BED around 200 Gy achieve the highest negative biopsy results. Back-converting the BED to the physical dose (using an α/β of 2) a BED of 200 Gy 2 can be achieved with postimplant D90s for 125 I of 188 Gy, 103 Pd of 167 Gy, and combination therapy with ERBT (25 fractions of 1.8 Gy) for 125 I of 110 Gy and for 103 Pd of 102 Gy.
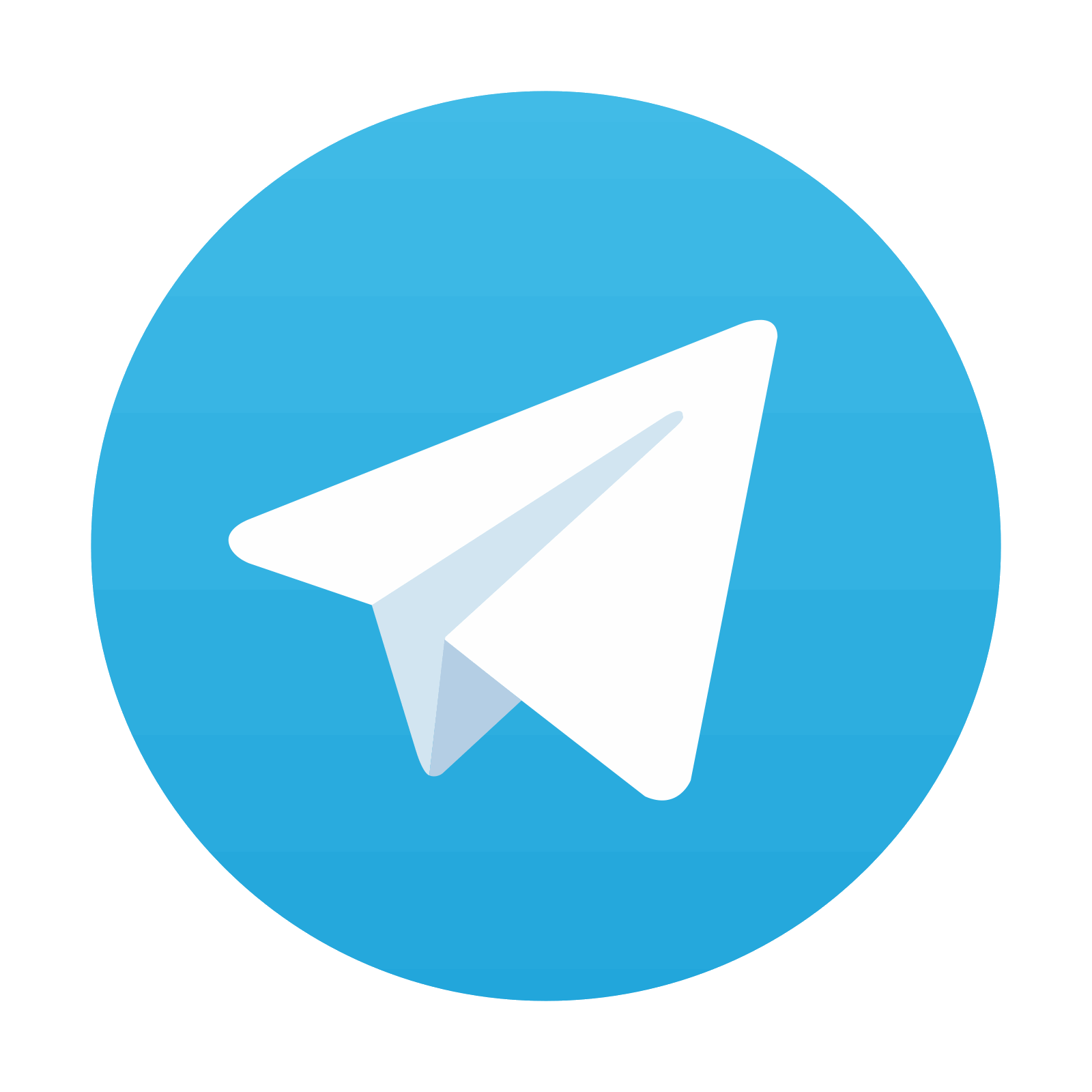
Stay updated, free articles. Join our Telegram channel
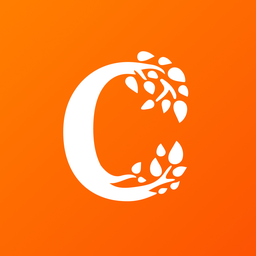
Full access? Get Clinical Tree
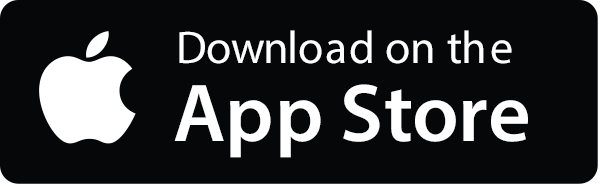
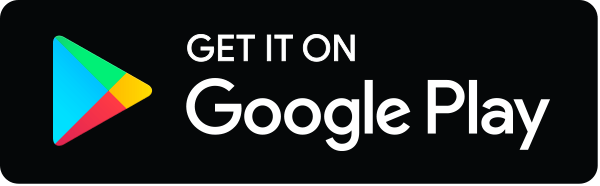