Abstract
Acute kidney injury (AKI) is a complex syndrome associated with several etiologic factors, including specific kidney diseases (e.g., acute interstitial nephritis [AIN] and acute glomerular diseases) and nonspecific conditions (e.g., ischemia, toxic injury). AKI occurs in a variety of settings with clinical manifestations ranging from a small elevation in serum creatinine (sCr) to anuric kidney failure. More than one etiologic factor may coexist in the same patient, and the manifestations and clinical consequences of different causes of AKI can be indistinguishable. The development of a uniform definition of AKI provided a better understanding of AKI incidence and its short- and long-term effects (Fig. 31.1). Epidemiologic studies over the last 10 years have shown that AKI nonrecovery is frequent, and chronic kidney disease (CKD) progression after an AKI episode arises as a main complication. Despite the advance in supportive therapy, treatment of AKI is largely dependent on the clinical presentation and the underlying etiology. In this chapter, we will focus on the tools available for risk stratification and detection of AKI.
Keywords
acute kidney injury, epidemiology, AKI definition, serum creatinine, glomerular filtration rate, renal recovery
Pathophysiology
The main causes of acute kidney injury (AKI) are associated with decreased kidney perfusion resulting from conditions such as hemorrhage, vomiting, diarrhea, poor oral intake, burns, kidney losses, impaired cardiac output, decreased vascular resistance, and renal vasoconstriction. Because the kidneys receive up to 25% of the cardiac output, any decrease in mean arterial pressure may affect kidney perfusion. A decrease in oxygen delivery severe or prolonged enough to impair cellular function can cause tubular or vascular endothelium dysfunction. The mismatch between oxygen delivery and demand is more prominent in certain regions of the kidney because variations in blood flow are characteristic of renal circulation. Acute tubular necrosis (ATN) describes the pathologic result from severe or prolonged hypoperfusion or toxic injury, but it is often not present in a patient with AKI. The most common findings described in AKI—detachment of renal tubular epithelial (RTE) cells from the basement membrane, effacement and loss of brush border in proximal tubular segments, formation of tubular casts derived from sloughed cells, tubular debris, interstitial edema, and congestion of the peritubular capillaries—are not necessarily present in human biopsy sample. In addition, the degree of glomerular filtration rate (GFR) falls and these pathologic alterations in AKI are often not correlated, demonstrating the complex interplay of vascular and tubular processes that determine kidney dysfunction in AKI.
The classification of AKI into initiation, extension, maintenance, and recovery phases can be helpful for understanding the interactions of inflammation and tubular and vascular factors in determining the extent of injury. The initiation phase occurs when renal blood flow decreases to a level that results in ATP depletion, or a toxin induces acute cell injury and/or dysfunction. The severity and duration of ischemia or toxic damage define the degree of the endothelial and/or tubular cell dysfunction. During this initiation phase, upregulation of cytokines triggers the inflammatory cascade, further worsening kidney perfusion. In addition, several endothelial mechanisms may affect kidney perfusion, including the regulation of intrinsic vascular constriction and response to vasoconstrictors or vasodilators. In the extension phase, the subsequent necrosis and apoptosis defines the extent of injury and fall in GFR. Although the inflammatory cell infiltration can be detected within 24 hours following ischemia and leukocytes may appear within 2 hours, this period is the most favorable phase to allow a therapeutic intervention to contain the amplification of the inflammatory response. In the maintenance phase, blood flow normalizes, GFR stabilizes, and the process of cell repair, migration, and proliferation initiates. In the following recovery phase, cellular differentiation continues and epithelial polarity is reestablished.
The degree of cell injury and dysfunction is heterogeneous in the kidney. While cells in the cortical region can remain viable and able to functionally and structurally recover, cells within the proximal tubule and the thick ascending limb in the outer medulla are more likely to suffer lethal injury. With sublethal injury, disruption of the actin cytoskeleton and loss of polarity lead to tubular back leak and obstruction. In more severe AKI, cell death occurs and can be confirmed by the presence of cellular debris and casts on urine microscopy. Two processes are associated with cell death; necrosis results in a profound inflammatory response as a trigger, while apoptosis initiates a regulated program leading to DNA fragmentation and cytoplasmic condensation without triggering an inflammatory response.
The remarkable efficiency of the reparative process after acute injury is attributable to the capacity of tubular epithelial cells to redifferentiate and restore the functional integrity of the kidney. New techniques of functional genomics and complementary DNA microarray have been able to demonstrate the complex pathways leading to kidney repair. Some of the genes upregulated in the reparative process are involved in cell-cycle regulation, inflammation, cell death regulation, and growth factor or cytokine production. The identification of these genes led to the discovery of early biomarkers of AKI, such as KIM-1, neutrophil gelatinase–associated lipocalin (NGAL), tissue inhibitor of metalloproteinase 2 (TIMP-2), insulin-like growth factor binding protein 7 (IGFBP7), and interleukin 18. In addition to their value for early AKI detection, these new biomarkers may also play a role in the process of injury and/or repair after AKI.
Acute Kidney Injury Definition
With the development of the classification system for AKI (RIFLE [Risk, Injury, Failure, Loss, and End-stage Kidney], AKIN [Acute Kidney Injury Network], and KDIGO [Kidney Disease International Global Outcomes]) and the introduction of the term AKI, we have seen an improved and broadly used definition of kidney injury ( Fig. 31.1 ). The RIFLE criteria were initially proposed in 2004, with AKIN criteria released in 2007 and the KDIGO system in 2012. The criteria maintain a 48-hour time interval for absolute changes in creatinine, and a 7-day time interval for relative changes in creatinine. The KDIGO group has also proposed a new term, acute kidney disease (AKD), to address the problem that changes in creatinine may evolve over periods longer than 7 days and thus not meet the AKI definition. AKD can occur with or without other acute or chronic kidney diseases (CKDs) and disorders (see Fig. 31.1C ).

Although the use of standardized definitions has greatly improved the current knowledge of AKI, there are still shortcomings with the criteria to define AKI. Serum creatinine (sCr) requires comparison to a baseline or a reference value that may not always be available. Thus to define AKI, we need two sCr values, including a “baseline” creatinine value representing the patient’s underlying kidney function. In the absence of a known baseline value, a reference value after injury initiation and loss of kidney function can delay or prevent AKI detection. Conversely, a reference value days after the hospital stay affected by possible loss of muscle mass can falsely define AKI.
Tools for Diagnosis, Staging, and Evaluation of Acute Kidney Injury
Standard Lab Tests
Serum Creatinine
Although the use of creatinine to assess kidney function is not ideal, it is widely available, easy to measure, and has been used for more than 80 years. Many factors other than kidney function, such as age, sex, muscle mass, catabolic rate, and race, influence creatinine concentration. sCr has a stable concentration, which makes it inadequate to access kidney filtration, a parameter that fluctuates continually. Thus changes in GFR often correlate poorly with changes in sCr concentration. Three factors influence the sCr concentration: the actual GFR, fluctuations in creatinine production, and fluid balance affecting the volume of distribution. Furthermore, in patients with normal kidney reserve, sCr may not change, despite acute tubular injury, because of compensatory increases in function of other nephrons, creating a delay in diagnosis after injury.
In AKI, sCr generation is not equal to filtration and excretion, resulting in retention of creatinine with a rising plasma level. Until the levels of creatinine reach a plateau at a new steady state, usually 24 to 72 hours after a known injury event, assessment of sCr can overestimate kidney function. Fluid administration is another common factor delaying AKI diagnosis by sCr, as fluid accumulation increases total body water (TBW), altering the volume of distribution of creatinine and resulting in potential overestimation of kidney function ( Fig. 31.2 ). The masking of AKI severity by volume expansion may be especially problematic in settings where the creatinine is rising relatively slowly because of either lower creatinine generation, as might be expected in the elderly or patients with less muscle mass, or to more modest overall injury. Analysis of the Fluids and Catheters Treatment Trial (FACTT) noted that sCr values corrected for fluid accumulation identified a larger number of patients with AKI who had been misclassified. These patients had outcomes similar to those with AKI.

Standardized creatinine assessments with the isotope dilution mass spectrometry (IDMS) method will improve the comparison across values and will improve agreement between values across centers. However, standardizing the assays has no effect on the individual variation of sCr. A normal sCr is frequently associated with low creatinine clearance (CrCl), especially in critically ill patients.
Creatinine Clearance
In critically ill patients with unstable kidney function in the nonsteady state, the loss of kidney function does not correspond to the degree of decline in estimated GFR. In those patients, the repeated measurement of CrCl may be an early indicator of AKI. For adjustment of medications, especially for toxic, antimicrobial, and chemotherapy drugs, it is fundamental to have a more reliable and accurate estimation of kidney function ( Table 31.1 ). In those circumstances, it is necessary to consider a CrCl, which can be performed in collection periods from 1 to 24 hours. The longer the collection time, the higher the likelihood for errors caused by inaccurate time recording and incomplete urine collection. Several studies have shown that short duration (1 to 4 hours) CrCl measurements are feasible in the critically ill, and studies have validated the method compared with 24-hour clearance. The use of 4-hour CrCl in the detection of AKI is also a valuable method.
Clinical Decisions | Change in Level of GFR |
---|---|
Diagnosis | Extremes of age (elderly, children) |
Extremes of body size (obesity, type 2 diabetes, low body mass index <18.5 kg/m 2 ) Severe malnutrition (cirrhosis, end-stage kidney disease) Grossly abnormal muscle mass (amputation, paralysis, myopathy) High or low intake of creatinine (vegetarian diet, dietary supplements) Pregnancy | |
Management | Dose and monitoring for medications cleared by the kidney |
Determine safety of diagnostic tests or procedures | |
Referral to nephrologists | |
Referral for kidney transplantation | |
Placement of dialysis access |
Commonly used formulas to estimate GFR are the Cockcroft-Gault and the abbreviated version of the Modification of Diet in Renal Disease (MDRD). Particularly in older, underweight, or overweight people, these equations overestimate GFR. The CKD-Epidemiology Collaboration (EPI) equation performs better in these patients. The Jelliffe equation was developed to estimate GFR in nonsteady-state conditions; it accounts for the difference between creatinine production and excretion, using the daily changes in sCr. Creatinine production is adjusted for age and for CKD status because creatinine production generally decreases with declining kidney function. The modified Jelliffe equation accounts for the effect of positive fluid balance in variations of sCr and correlates best with measured CrCl.
Blood Urea Nitrogen
Blood urea nitrogen (BUN) is also used to evaluate kidney function because elevations in BUN level are often, but not always, due to a GFR decrease. Some conditions enhance urea production, such as gastrointestinal bleeding, corticosteroid therapy, and high-protein diet. In the noncatabolic patient with mildly reduced GFR, daily BUN usually increases less than 10 to 15 mg/dL/day, whereas high catabolic states and high-protein diets are associated with greater urea nitrogen production that can exceed 50 mg/dL. In the presence of a decreased intravascular effective volume, BUN increase is not proportional to the rise in sCr level and fall in GFR. Normally, the BUN:sCr ratio is about 15 : 1, with the BUN and sCr increasing by 10 to 15 and 1.0 to 1.5 mg/dL/day, respectively, in the absence of glomerular filtration. In situations characterized by decreased glomerular perfusion pressure such as heart failure, BUN can increase independently from sCr.
Cystatin C
Cystatin C is an alternative blood marker that increases as GFR decreases. In CKD, it is an alternative to sCr for estimating GFR. Factors other than GFR affect cystatin C levels, such as glucocorticoid use, thyroid status, cancer, obesity, diabetes, and inflammation. Cystatin C has one-third of the volume of distribution of creatinine, thus reaching a new steady state three times faster than creatinine. This has the potential of predicting AKI before sCr increases. However, cystatin C and sCr have the same limitations associated with positive fluid balance.
Urine Studies
Urine Flow: Oliguria
The normal response of the kidney to decreased effective intravascular volume and kidney perfusion is to concentrate the urine and increase sodium reabsorption. As such, AKI associated with intravascular volume depletion is often accompanied by oliguria, and urinary flow can provide helpful information about the cause of AKI ( Table 31.2 ). However, several other factors affect urine flow, including volume expansion, high-dose potent diuretic agents, and renal vasodilators. Although oliguric AKI is more frequent, nonoliguric AKI occurs in approximately 33% of cases at diagnosis. Nonoliguric states may be present in all types of AKI, including those following surgery, trauma, hypotension, nephrotoxins, and rhabdomyolysis.
Performance Measure | FE Na (<1% or >3%) | FE Urea (<35%) |
---|---|---|
Sensitivity | ||
For prerenal azotemia | 78–96% | 48–92% |
For prerenal azotemia on diuretic | 29–63% | 79–100% |
For intrinsic causes | 56–75% | 68–75% |
Specificity | ||
For prerenal azotemia | 67–96% | 75–100% |
For prerenal azotemia on diuretic | 81–82% | 33–91% |
For intrinsic causes | 78–100% | 48–98% |
Positive Predictive Value | ||
For prerenal azotemia | 86–98% | 79–100% |
For prerenal azotemia on diuretic | 86–89% | 71–98% |
For intrinsic causes | 64–100% | 43–94% |
Negative Predictive Value | ||
For prerenal azotemia | 60–86% | 43–83% |
For prerenal azotemia on diuretic | 18–49% | 44–83% |
For intrinsic causes | 82–86% | 79–86% |
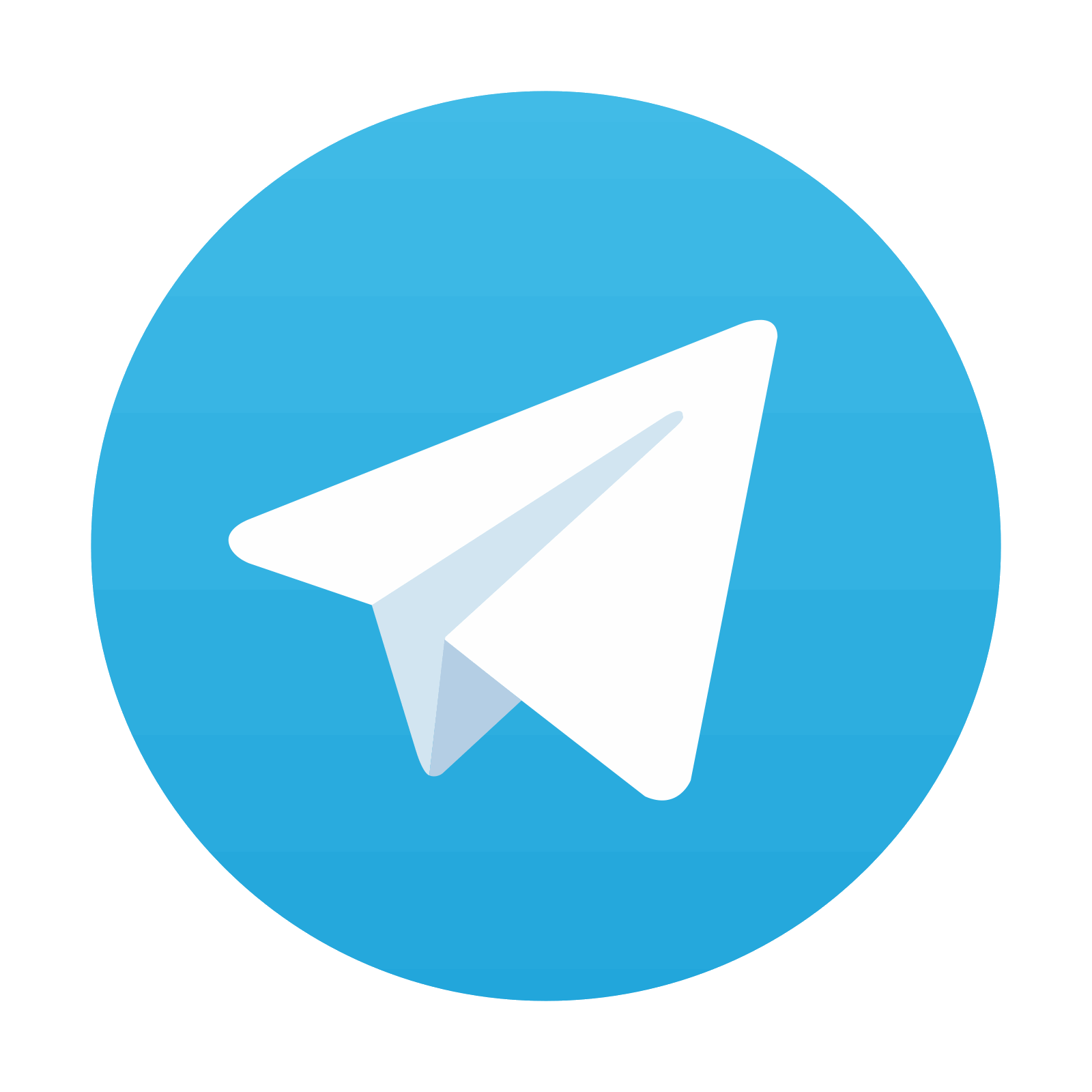
Stay updated, free articles. Join our Telegram channel
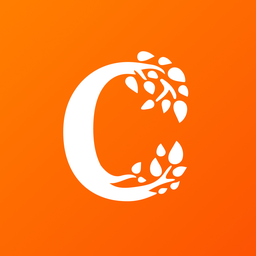
Full access? Get Clinical Tree
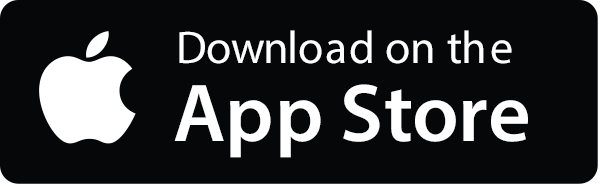
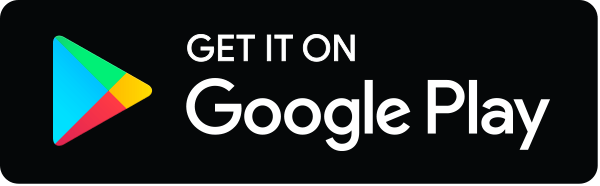
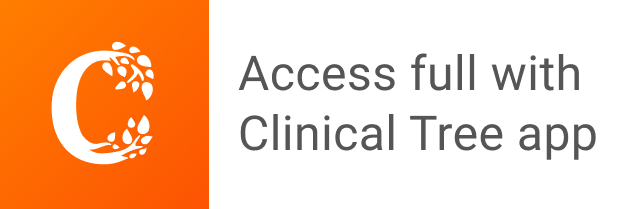