© Springer International Publishing Switzerland 2017
Giancarlo Balercia, Loredana Gandini, Andrea Lenzi and Francesco Lombardo (eds.)Antioxidants in AndrologyTrends in Andrology and Sexual Medicine10.1007/978-3-319-41749-3_22. Biochemistry of Coenzyme Q10
(1)
Department of Clinical and Dental Sciences – Biochemistry Section, Polytechnic University of Marche, Ancona, Italy
2.1 Introduction
Coenzyme Q (CoQ) is a lipophilic quinone widely distributed in nature. The first homologue to be discovered about 50 years ago, in beef mitochondria, was coenzyme Q10 by Dr. P.L. Crane [8]. From those early studies, the essential coenzymatic activity in mitochondrial bioenergetics was soon recognized. CoQ is made of benzoquinone moiety and an isoprenoid side chain, the length of which is 10 units both in man and many mammals. Other living organisms possess different species of CoQ, for instance, Saccharomyces cerevisiae produces CoQ6, other microorganisms CoQ7 and many mammals CoQ9. Each organism possesses a dominant homologue of CoQ and minor amounts of other homologues; differently from other isoprenylated compounds introduced in the diet, such as menaquinones, the elongation of a certain CoQ homologue to CoQ10 is not possible. Most of the coenzyme Q10 in our organism is endogenously produced, although a small amount is ingested daily through the diet where it is present mainly in meat and fish and to a lower extent in some vegetables for an average intake of 5 mg/day. For this reason, being an endogenous cofactor
and hence not essential, but partially taken with the diet, it is also referred as a vitamin-like molecule.
Coenzyme Q10 is also known as ubiquinone because of its widespread, ubiquitous diffusion in living organisms [29]. This name was coniated by Dr. R.A. Morton, who also isolated and studied the compound in the early years of its discovery.
For a certain number of years, CoQ was mainly known for its key role in mitochondrial bioenergetics; later studies demonstrated its presence in other subcellular fractions and in plasma and extensively investigated its antioxidant role. The rationale supporting the use of CoQ10 as a food supplement is mainly based on these two functions. At the inner mitochondrial membrane level, ubiquinone is also recognized as a cofactor for the function of uncoupling proteins and a modulator of the transition pore [9]. More recent data reveal that CoQ10 affects the expression of genes involved in human cell signalling, metabolism and transport [15], and some of the effects of exogenously administered CoQ10 may be due to this property. New progress has been made in elucidating CoQ10 in metabolism and nutrition. This short chapter focusses on basic biochemical mechanisms of CoQ10 in order to provide a background to better understand the relationship between those and physiological and clinical effects, with particular relevance to reproductive health.
2.2 CoQ10 and Mitochondrial Bioenergetics
The discovery of the CoQ10 molecule came about as a result of an intensive programme of research developed by Prof. D.E. Green, at the University of Wisconsin, to find out how mitochondria work. This occurred in 1957. It soon became evident [14] that coenzyme Q was essential to mitochondrial ATP formation, i.e. to the most efficient mechanism which leads to the release of chemical energy housed in our nutrients. Several years later, Prof. P. Mitchell was awarded the Nobel Prize, for his studies centred on the vital role of coenzyme Q in oxidative phosphorylation. According to this early view, the quinone was considered as a substrate in excess concentration over the prosthetic groups in the lipoprotein complexes of the respiratory chain. The kinetic analyses of Kroger and Klingenberg [19] showed that steady-state respiration in submitochondrial particles could be represented as a simple combination of two enzymes, the first one responsible for the reduction of coenzyme Q and the second one causing oxidation of ubiquinol. Experiments of direct titrations of CoQ-depleted mitochondria reconstituted with different CoQ supplements disclosed a Km of NADH oxidation close to a CoQ concentration of 4–10 mM in the lipid bilayer, whereas the Km for succinate oxidation was one order of magnitude lower [12]. The physiological concentration therefore is not saturating, and even a small increase in the CoQ10 concentration of mitochondrial membranes could lead to an increased respiratory rate; on the other hand, even small decreases in cellular CoQ10 content may translate in remarkable impairment of mitochondrial functionality. The control exerted by CoQ concentration over mitochondrial respiration could be of particular interest in situations of decreased CoQ levels and/or increased Km values for the quinone in some pathological states. In fact this observation could represent the biochemical mechanism by which exogenous coenzyme Q10 ameliorates the bioenergetic impairment in some mitochondrial myopathies and in cardiomyopathy [28, 31]. Modern views indicate that respiratory complexes may have a supramolecular organization, i.e. stable super complexes [13]. The advantage of this super complex organization would be a more efficient electron transfer by channelling of the redox intermediates. Preliminary data suggest that alteration of the protein/phospholipid ratio and lipid peroxidation disaggregates the supercomplex organization with possible pathophysiological implications. Lenaz postulates [13] that in ageing, and in ischemic diseases, reactive oxygen species (ROS) produced by the mitochondrial respiratory chain induce a progressive peroxidation of mitochondrial phospholipids. This could lead to a dissociation of complex I–III aggregates and subsequent loss of facilitated electron channelling. Also according to this model, an increased concentration of coenzyme Q may, at least partially, counteract this deleterious effect of supercomplex disaggregation.
CoQ as an Antioxidant
In its reduced form (ubiquinol), coenzyme Q acts as a phenolic antioxidant, undergoing hydrogen abstraction by free radicals; therefore, it acts like a chain-breaking antioxidant. This evidence has been produced by numerous experimental models, both in vivo and in vitro, using artificial membranes, isolated subcellular organelles, cultured cells, isolated perfused organs and clinical models. Lars Ernster, a pioneer of the studies on the location and the function of coenzyme Q in mitochondrial membranes, reviewed the mechanisms by which coenzyme Q, mainly in its reduced form, is currently believed to exert its antioxidant effect [11]. Experimental data strongly indicate that the intervention of coenzyme Q, in its reduced form, can be considered within the antioxidant preventive mechanisms. CoQH2 would reduce the perferryl species (Fe+++ − O2 •−), thus preventing the radical attack that this species would initiate on fatty acids. By this mechanism, QH2 would practically prevent the formation of alkyl radicals (L•) and peroxyl radicals (LOO•). Ubiquinol may also act by slowing down the chain propagation reaction, according to the general mechanism of hydrogen donation to the radicals. Another mechanism by which ubiquinol exerts its antioxidant properties is the one explored by Packer, Kagan, et al. [17]. These authors demonstrated that reduced coenzyme Q regenerates α-tocopherol, the active form of vitamin E, by reducing the α-tocopherol radical. In these reactions, the ubisemiquinone radical is formed, whose presence in the mitochondrial respiratory chain has been known for the past 20 years. Ubisemiquinone is stabilized through the binding to special Q proteins. The presence of SOD and catalase would keep under control O2 •− and the H2O2 arising from the autoxidation of the ubisemiquinone; however, it is unlikely that autoxidation of the ubisemiquinone species generates O2 •− in the phospholipid environment. Ernster stresses the fact that coenzyme Q is the only a lipid-soluble antioxidant that animal cells can biosynthesize de novo and for which appropriate enzymatic mechanisms exist to regenerate the reduced form. We have demonstrated that reduced coenzyme Q exerts its antioxidant properties also by inactivating ferrimyoglobin, a species capable of triggering the oxidative attack at muscular and cardiac level [27]. Oxidative stress can of course attack the plasma membrane, which delimits the cell environment and also constitutes a protection against different kinds of oxidative insult from the environment. Different antioxidants are responsible for this protection, in particular ascorbate on the hydrophilic cell surface and CoQ, together with α-tocopherol in the hydrophobic phospholipid bilayer. In order to act as an antioxidant, CoQ must be in the reduced state; several enzymes exert this function of CoQ reductases. NADH-cytochrome b5 reductase and NQO1 are among the principal reductase systems [32]. Numerous data indicate that the plasma membrane redox system plays an important role in preserving the antioxidant capacity during oxidative stress insults related both to the diet and to the ageing process. It is known that caloric restriction (CR) can attenuate oxidative stress and age-related oxidative stress. Plasma membrane redox system, of which CoQ is an essential constituent, is influenced by CR also through CoQ intervention. It was found that CoQ-dependent NADPH dehydrogenases were increate in plasma membranes from aged rats treated with caloric restriction [10]. As a consequence, the liver plasma membranes of aged rats treated with CR were more resistant to oxidative stress-induced lipid peroxidation compared to the membranes from rats fed ad libitum. Therefore, the antioxidant system in the plasma membrane is involved in the healthy ageing induced by CR. Also the plasma membrane content of CoQ, which declines with age, is enhanced by CR [32]
.
2.3 Endogenous and Nutritional Sources of Coenzyme Q10
Coenzyme Q10 is widely diffused in nature; thus, it is present in many vegetal and animal tissues which are part of our normal diet. Coenzyme Q10 is also actively synthesized by our cells. This is the reason it is not a vitamin according to the classical definition. So, our tissue levels of CoQ10 depend both on an endogenous biosynthesis and on an exogenous supply. Metabolic demand and the turnover rate of CoQ10 should also be taken into consideration when trying to establish a “nutritional status” of CoQ10. Nutritional intake: the content in CoQ9 and CoQ10 of different types of food was evaluated in a paper by [18]. CoQ9 is usually present in cereals, while CoQ10 is the ubiquinone of soybeans. CoQ10 is also present in walnuts, almonds, oils, fruits rich in oil and green vegetables; spinach is particularly rich in CoQ10. Some kinds of fish also have comparatively high amounts of CoQ10. On a weight basis, sardines have more than twice as much CoQ10 as beef; 1.6 kg of sardines contain 100 mg of CoQ10. Milk and cheese have a lower content of coenzyme Q10. It is difficult to assess the relative importance of endogenous biosynthesis and exogenous intake; the latter plays a significant role as well. Data from Kishi et al. (1986) showed that in patients under total parenteral nutrition (TPN), plasma levels of CoQ10 undergo a remarkable reduction (50 %) in just 1 week. This finding might be related to non-consumption of CoQ10 and/or its precursors present in the diet or to stressors that resulted in the patient requiring TPN. In our lab we often found plasma levels of CoQ10 close to or even lower than 0.1 μg/ml in traumatized patients. It is difficult to evaluate to what extent those data were related to TPN or to the serious clinical conditions of shocked patients. 0.1 μg/ml represents a very low value in as much as normal plasma levels are around 0.79 ± 0.2 μg/ml. Blood CoQ10 is mainly transported by LDL, although it is also present in the other classes of lipoproteins and in blood cells [49]. Its concentration is usually reported in micrograms/litre of plasma or micromoles/litre. But it is worthwhile to normalize these values according to the blood LDL content or at least to plasma cholesterol levels. The CoQ10/total cholesterol level could have a predictive value in cardiovascular disease as discussed below.
The endogenous synthesis of the quinone moiety of CoQ10 in our organism starts from phenylalanine or from tyrosine and the isoprenoid side chain derives from mevalonate. A series of vitamin cofactors is needed for this biosynthesis. It was highlighted that in a vitamin B6 deficiency plasma CoQ10 levels are also low and they increase upon improvement of the vitamin B6 deficiency status [55]. In eukaryotes the isoprenoid side chain of coenzyme Q is synthesized through the mevalonate pathway. Mevalonic acid (MVA) is converted to isopentenyl diphosphate (IPP). Dimethylallyl diphosphate acts as a primer and is progressively elongated by IPP to construct isoprenoid chains with progressively increasing length. Addition of a new isoprene to the growing polyisoprenoid chain can occur only at the alpha end since the process is energy requiring and there must be a leaving pyrophosphate group. Consequently, it is not possible to add a new isoprene to the omega end. During CoQ synthesis, solanesyl-PP or decaprenyl-PP is attached to the benzoquinone ring at the alpha end by the help of the leaving PP. The free end of the solanesol is the omega end without PP, and we do not know any enzymatic reaction which can mediate the addition of an additional isoprene. From a practical point of view, this implies that CoQ9, or a shorter homologue, taken with diet, cannot be elongated to CoQ10. As pointed out by [4, 5], dietary administration of CoQ9 or CoQ10 results in a number of metabolites which influence the endogenous synthesis of CoQ. In this respect, administration of CoQ9 may increase CoQ10 synthesis [4, 5].
Statins and CoQ10
Statins are 3-hydroxy-3-methylglutaryl coenzyme A (HMG-CoA) reductase inhibitors which decrease synthesis of mevalonate, a key metabolic step in the cholesterol synthesis pathway. These efficient drugs can produce a variety of muscle-related complaints or myopathies. Since the mevalonate pathway also leads to the biosynthesis of the isoprenoid side chain of coenzyme Q10, different studies have addressed the possibility of CoQ10 being an etiologic factor in statin myopathy. This issue has been extensively investigated, and it is worthwhile to mention two reviews by Littarru and Langsjoen [23, 24]. It was highlighted that, besides decreasing plasma CoQ10 levels, statin treatment also leads to lower lymphocyte levels of CoQ10. There are no univocal results about the effect of statin treatments on CoQ10 levels in skeletal muscle [21, 20], yet more recently [38] it was reported that high-dose statins did decrease muscle CoQ10 and mitochondrial respiratory chain activities, possibly related to reduction in the number or volume of muscle mitochondria. In a 2008 study, an inverse correlation between atorvastatin-induced changes in CoQ10 and BNP was found. It was concluded that long-term treatment with atorvastatin might increase plasma levels of BNP in patients with coronary heart disease when accompanied by a greater reduction in plasma CoQ10 [46]. Regarding the effect of CoQ10 supplementation, this was found not to improve statin tolerance or myalgia in one study [57], whereas [7] reported a positive effect of CoQ10 on pain severity and pain interference in daily activities in a group of statin-treated patients showing myopathic symptoms. A larger clinical trial where patients suffering from some statin side effects are treated with CoQ10 would shed more light on this issue.
Human CoQ10 Deficiencies
Already in the past CoQ10 had been shown to be effective in a number of cases of mitochondrial myopathies, which were sometimes associated with low CoQ10 muscle levels. With the progress in molecular biology techniques, primary CoQ10 deficiencies, due to mutations in ubiquinone biosynthetic genes, have been identified, and they are associated with four major clinical phenotypes [39, 40]. Some of these syndromes have shown excellent responses to oral CoQ10 treatment. It has been ascertained that respiratory chain dysfunction and oxidative stress correlate with the severity of primary CoQ10 deficiency [39, 40]. Not all conditions respond to CoQ10 administration, perhaps also on the basis of the time when CoQ10 therapy was started; some of the responsive cases also showed an improvement of a nephropathy [26, 43].
2.4 Clinical Applications of Coenzyme Q10
In light of the mechanisms described above, a wide range of pathophysiological conditions associated with an increase in oxidative stress have been shown to be related to decreased CoQ levels and/or increased ubiquinone/total CoQ10 ratio. These conditions include physiological conditions such as the ageing process [37], intense physical exercise as well as a wide range of clinical conditions such as metabolic diseases, cardiovascular disease [22] and neurodegenerative and genetic disorders [34, 35, 50]. A detailed description of the role of CoQ10 in these conditions is beyond the scope of this review that will be limited to a brief description of the role of CoQ10 treatment in cardiovascular disease in light of its relevance and association with reproductive health and fertility issues [16, 36, 41].
In fact cardiovascular disease probably represents the clinical field where the beneficial role of CoQ10 has been better described, and its effects on CoQ10 can be ascribed to its bioenergetic role, to its capability of antagonizing oxidation of plasma LDL and to its effect in ameliorating endothelial function.
In this context, support of mitochondrial bioenergetics is directly linked to myocardial contractility, while the latter two mechanisms are associated to the systemic antioxidant protection exerted by CoQ in the vasculature that has also direct important implication in reproductive health.
It is currently believed that high levels of LDL, as well as smoking and hypertension, are primary risk factors, among those contributing to cardiovascular disease. Biochemical mechanisms responsible for the atherogenicity of LDL have been extensively addressed, and experimental evidence has been produced indicating that oxidatively modified LDL become atherogenic. It was found that endothelial cells are involved in the oxidative attack against LDL [10] as described above. Oxidative attack on LDL deeply affects the apoprotein moiety as well. As a consequence of these changes, LDL are no longer “recognized” by the normal receptors and are taken up more readily by the scavenger receptors of macrophages. LDL leave the blood stream, penetrate the endothelial cell lining and reach the subendothelial space, where they undergo oxidative attack. Oxidatively modified LDL are capable of triggering further events, including platelet activation, and exert a chemotactic attraction on circulating monocytes, which migrate to the subendothelial space, where they become macrophages. These cells have only low levels of the classical LDL receptor; nonetheless, they are able to take up more rapidly oxidatively modified LDL, and this uptake involves a different receptor, called the “scavenger receptor”. As discussed above, oxidatively modified LDL are easily recognized by the scavenger receptors. These events lead to an accumulation of lipids, mainly cholesterol and cholesterol esters, in the macrophages, which will become lipid-laden foam cells. Foam cells may be considered the essence of the atheromatous lesions. LDL are endowed with a number of lipid-soluble antioxidants capable of preventing or minimizing lipid peroxidation.
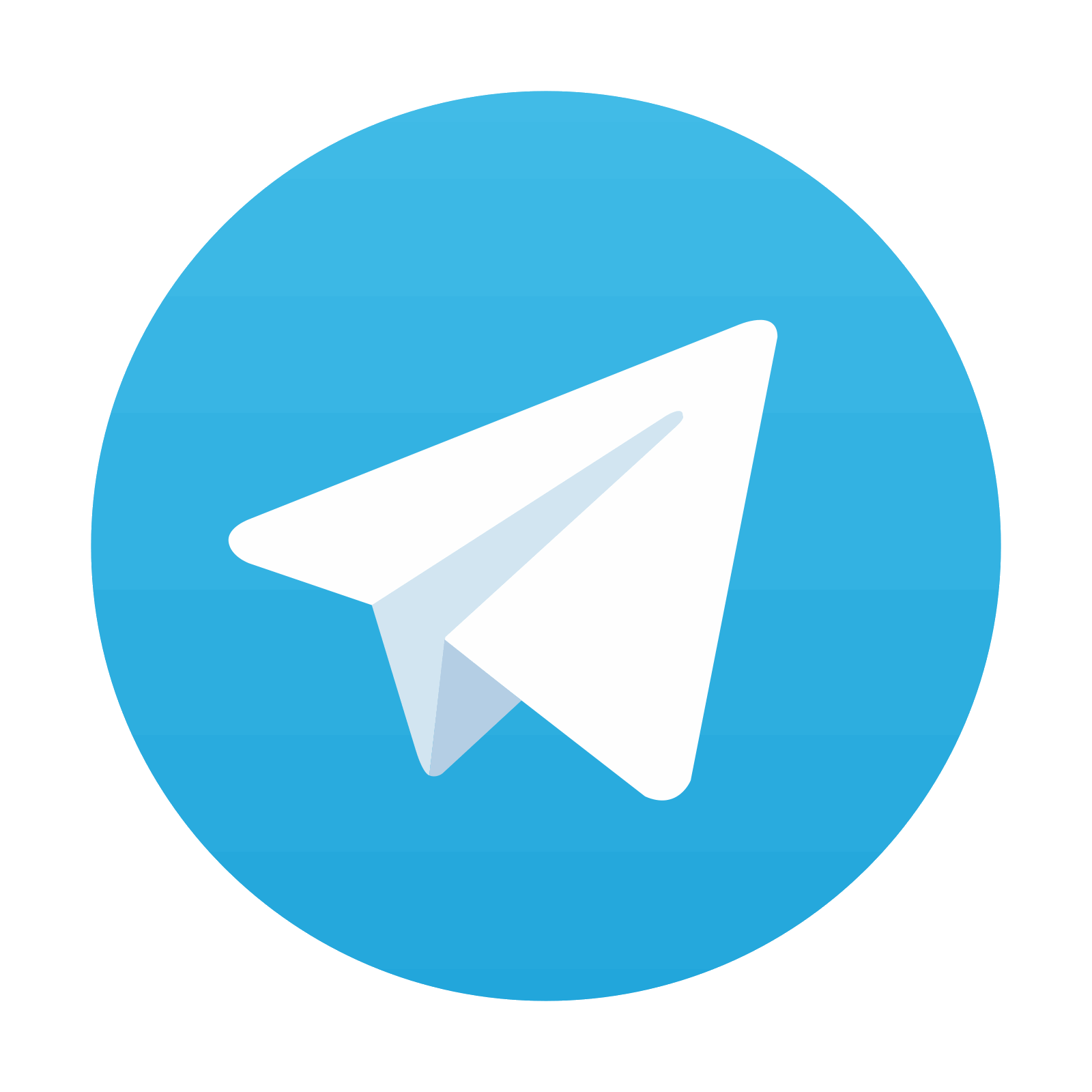
Stay updated, free articles. Join our Telegram channel
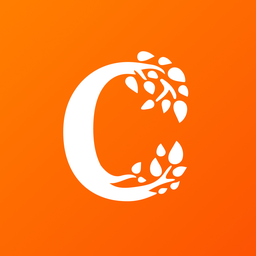
Full access? Get Clinical Tree
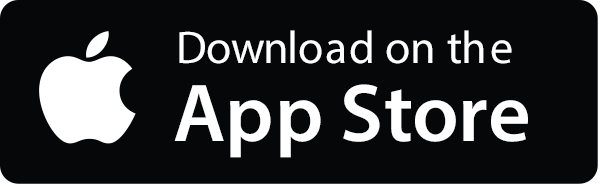
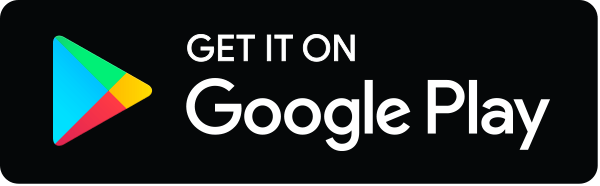