Evolution of the Robot: a Brief Journey Through Time
The Robot Institute of America (1979) defined the robot as “a reprogrammable multifunctional manipulator designed to move material, parts, tools or specialized devices through various programmed motions for the performance of a variety of tasks.” While the word “robot” is eponymous from the Czech author Karel Capek’s book Rossum’s Universal Robotics (RUR) in 1920, human fascination for robots and automatons actually goes centuries back ( ).
The first real foray of the Western world in the field of robotics was said to have been made by Leonardo da Vinci, whose drawings show vivid illustrations of mechanized armored knights, carts, and animals. From musical automatons, life-like toys, and mechanized animals during the latter half of the 2nd millennium, the evolution of robots took a decisive turn when von Kempelen, a Hungarian author and inventor, designed his sophisticated chess-playing machine The Turk in 1770 that played against, and beat, human beings. The idea of a robot executing tasks more efficiently than humans started taking shape. By the 20th century, robots were occupying the mindspace of Americans: Rossum’s RUR talked about robots (with its plot involving a farfetched-yet-sinister war with humanity), and Isaac Asimov presented his three laws of robotics in his famous book Runaround . Almost around the same time, the Eastern World, led by Japan, was exploring, developing, and perfecting humanoid robots (fueled by the cultural revolution brought about by the popular comic book character “Atom Boy”), the underlying theme being that robots are intelligent, worthwhile creations designed to help their human counterparts, in industrial and domestic settings alike.
The field of surgical robotics started in the United Kingdom, where Brian Davies, JEA Wickham, and a team of urologists developed a six-axis PUMA robot device in 1987, aptly called the PROBOT, for prostate surgery. In the United States, the genesis of robotic surgery was driven by the National Aeronautics and Space Administration, in collaboration with surgeons from the Stanford Research Institute, who put forth the fundamentals of telepresence surgery. By combining dexterity-enhancing robotic manipulation with the 3-D audio-visual “virtual reality” system, the motions of the surgeon’s fingers could be digitally tracked via “data gloves” and reproduced at the remote robotic instruments. This later formed the basis for the team at Intuitive Surgical, that ultimately went on to develop the da Vinci robotic system in 1999. The first clinical trial using the da Vinci robot was performed on a series of 200 patients undergoing robotic cholecystectomy, which led to the Food and Drug Administration (FDA) approval for the robot in July 2000.
Robotic Surgery: the Essentials
The da Vinci Surgical System
Starting from 1999, five different da Vinci systems have been released, each offering incremental tools for greater surgical dexterity. Given the realization that robotic surgery may be associated with a steep learning curve, the later versions of the da Vinci systems aimed to simplify manipulation while allowing greater surgeon autonomy. The latest version, da Vinci Xi, was launched in 2014 and various robotic centers across the world are currently in the process of upgrading to the Xi system.
The essential components of a robotic surgical system includes the surgeon console, the patient cart, the vision cart, and the EndoWrist instruments. We will briefly mention the salient features of each of these components; details regarding their functionality may be obtained elsewhere ( ).
- 1.
The surgeon console ( Fig. 7.1A ), as the name suggests, houses the master controls for the robotic system; it also allows a three-dimensional (3D) view of the surgical field through the stereoviewer and manipulation of the robotic instrument arms using the finger-controlled master controllers and foot pedals. The pod control buttons (located on the right and left in older systems and on the central touch pad in the Si) turn the system on–off, communicate system errors, troubleshoot faults, and adjust the height of the surgeon console for optimal ergonomic positioning. The master controllers ( Fig. 7.1B ) relay the movements of the surgeon’s hand, wrist, and fingers, after scaling (2 : 1, 3 : 1, or 5 : 1) and filtering any physiological tremors in the surgeon’s hand, producing precise, real-time movements of surgical instruments. The foot pedals control the position of the camera, instruments, toggling between coagulation/cut modes, and activating auxiliary visual channels (intraoperative ultrasonography, fluorescence imaging).
FIGURE 7.1
( A ) Surgeon console, da Vinci Si with ( B ) master controllers.
- 2.
The patient cart ( Fig. 7.2 ) is positioned close to the patient during surgery. It houses the camera and the three instrument arms; each arm has set-up clutch buttons to assist the movements of the arm, adjust its trajectory, and insert/withdraw instruments during docking/dedocking and in surgery. The movement of the surgeon’s fingers at the controllers are transmitted to the instruments that “operate” within the surgical field. Multiple sensors ensure a redundant safety-check system to prevent any independent movement of instruments or robotic arms.
FIGURE 7.2
Patient cart with camera arm and robotic arms, draped before surgery and after being docked in position ( inset ).
- 3.
The vision cart ( Fig. 7.3 ) comprises the cold light source (a xenon fiber-optic system), video-processing equipment, camera focus control, and camera storage bin; the key part of this system are the right and left optical channels from the endoscope that are connected to two 3-chip camera control units (CCUs), and the input from these CCUs are integrated to produce the high-definition 1080i (1920 × 1080 for Si systems) 3-D image at the surgeon stereoviewer. S systems and later versions also have a touch screen monitor (mounted on the patient or vision cart). The vision (or the patient) cart also has a mounted touch screen monitor, synchronized with the stereoviewer and displaying all the system status icons and messages. This touch screen system can be used for endoscope alignment (“white balance”), toggling between video inputs, or for telestration (allowing the surgeon or the team members to draw real-time images on the screen that can then be relayed to the stereoviewer, a feature that is useful for training of residents and fellows).
FIGURE 7.3
( A ) Vision cart with mounted touch screen monitor. ( B ) The touch screen monitor can be used for “telestration,” or drawing of real-time images on the screen that can be seen in the stereoviewer of the surgeon console to guide dissection ( arrow ).
- 4.
The EndoWrist instruments ( Fig. 7.4 ) are fit into the patient cart and translate the movements of the surgeon’s fingers, providing seven degrees of freedom (180 degrees of articulation and 540 degrees of rotation), far greater than the human hand, while eliminating surgeon hand tremors. The commonly used 8-mm instruments (shaft diameter 8 mm; Fig. 7.5 ) have an “angled” joint allowing the tip to rotate with a shorter radius compared to the 5-mm “snake” jointed ones.
FIGURE 7.4
A sample of available robotic instruments. Only 8-mm instruments are demonstrated. ( A ) Maryland bipolar: used as the primary instrument in surgeons non dominant hand. ( B ) Needle drivers: smaller pediatric needle drivers are available. ( C ) Prograsper: as the name implies, best instrument for grasping. Used during dissection of seminal vesicles, vas deferens dissection. Aids in retraction of bowel during radial cystectomy and partial nephrectomy. ( D ) Monopolar scissors: workhorse instrument used in all procedures in the surgeon’s dominant hand. Allows for cutting and coagulation. ( E ) Clip applier: excellent for precise placement of Weck Hem-o-lok Clips (Teflex, Research Triangle Park, NC) during nerve-sparing prostatectomy or during renal surgery. ( F ) Monopolar hook: May be used for dissection near vessels during pelvic lymph node dissection or renal hilum. ( G ) Cold scissors: for athermal cutting and dissection, such as during nerve-sparing radical prostatectomy. ( H ) Robotic stapler: for control of large vessels, such as hilar vessels during radical nephrectomy. ( I ) Robotic vessel sealer.
FIGURE 7.5
EndoWrist needle drivers. Left , 5-mm needle driver with “snake joint.” Right , 8-mm needle driver with “angled joint.”
(Adapted, with permission, from Higuchi T, Gettman M. Robotic Instrumentation and Operating Room Setup. In: Hemal A, Menon M, editors. Robotics in Genitourinary Surgey. 1st ed. New York: Springer; 2011. p. 25-36.)
Operating Room Setup
Essentially, four key requirements should guide the setup of the operating room (OR) for robotic surgery:
- 1.
Clear, unobstructed view of the patients from the surgeon console
- 2.
Tension-free cable connections between different pieces of equipment
- 3.
Clear paths and spaces for OR personnel for free movement and maneuvering within the OR.
- 4.
Facilitate “docking” of the robot from different angles (such as side-docking or end-docking), the need for which may be crucial for certain surgeries.
A schematic and actual representation of a robotic OR is illustrated in Fig. 7.6 . Note that modern-day robotic ORs may have additional features such as an external recording system (for recording surgical videos), connections to external displays (for projecting the view in surgeon’s console to secondary monitors at remote locations, such as during webcasting a live surgery), ceiling-mounted flat panel monitors, and equipment booms for housing insufflators, electrosurgical units, laparoscopic camera equipment, and light sources.
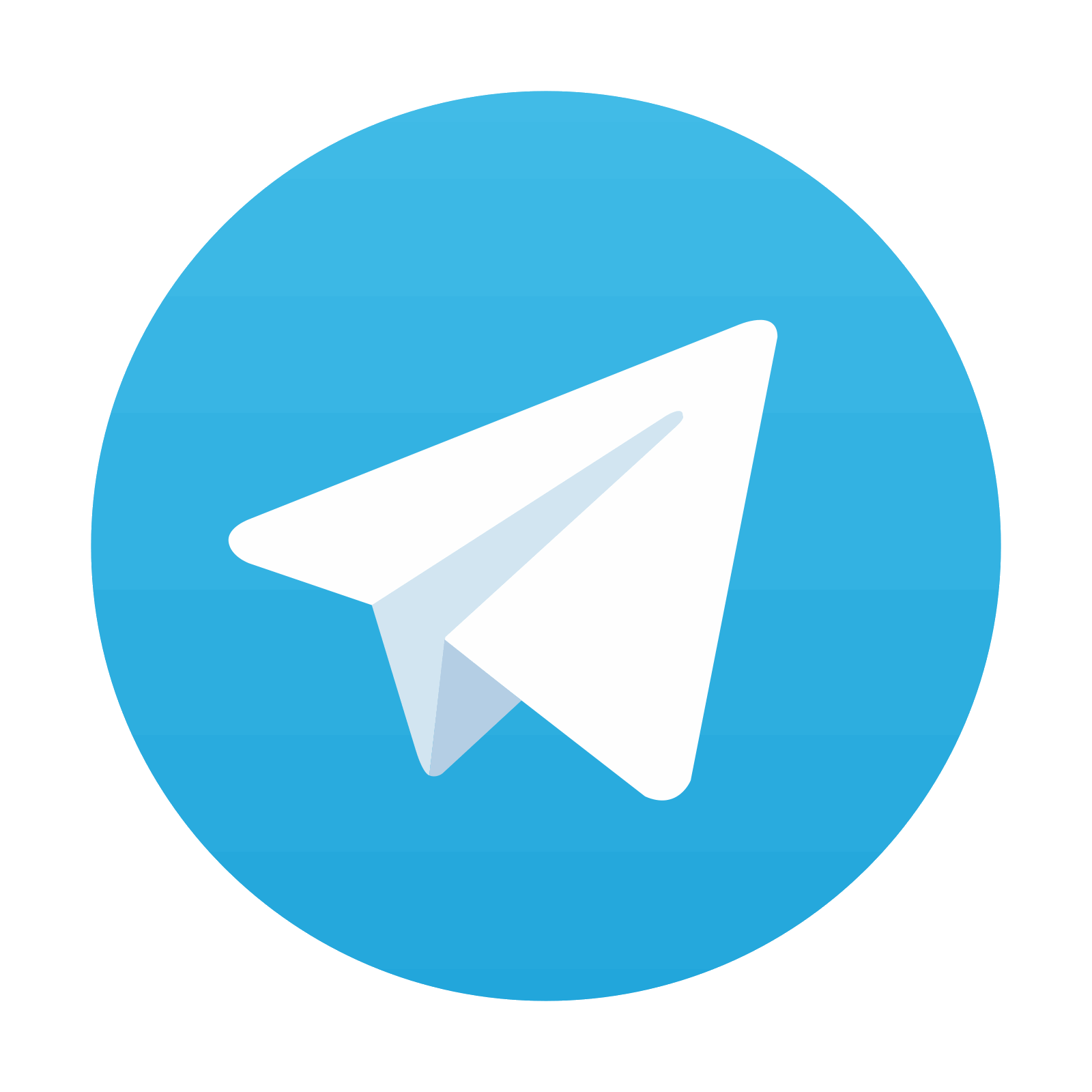
Stay updated, free articles. Join our Telegram channel
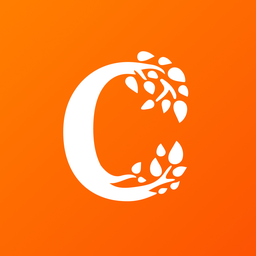
Full access? Get Clinical Tree
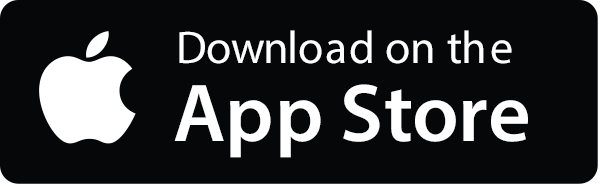
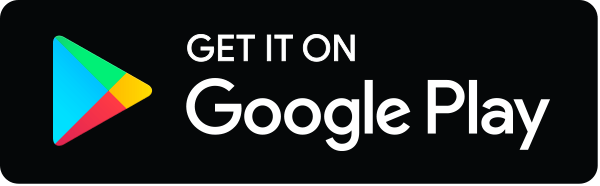
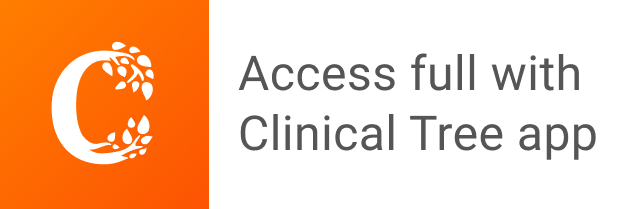