The gastrointestinal tract handles our daily vital task of safely receiving and extracting nutrients from what we eat and drink. Our gut is efficient because of a complex, coordinated, and adaptable system, although it is not without limits. A significant cumulative volume of fluids from the aerodigestive tract rich in electrolytes, proteins, and bile acids is recycled daily, minimizing waste as feces. The gut is also home to our flora including bacteria, viruses, and yeast with their massive DNA and RNA pool that dwarfs our very own. The lively interactions between our flora and our immune system can determine health and disease states. Our digestive physiology offers a fascinating glimpse into the story of our own evolution as our ancestors faced environmental and dietary changes. It also is intricately associated with the history of cooking, which may have influenced our anatomic and social evolution.
A congenital or acquired alteration in the physiology of the gastrointestinal system can result in significant morbidity and mortality. An understanding of the different aspects of digestion and absorption helps us appreciate how disease states occur and how they can be managed. By utilizing some of the known concepts of electrolyte absorption, with oral rehydration salts, we have decreased mortality from acute diarrhea from 5 million to 1.3 million deaths annually.
Key Principles of Digestion and Absorption
The main components of our diet are water, electrolytes, carbohydrates (starches and sugars), proteins, fats, nucleic acids, vitamins, and minerals. It is important to consider that we do not consume peptides, triglycerides, and disaccharides as such, but rather we eat meat, oils, and fruit, to name a few examples. Our nutrients come packaged with a structural complexity that affects the body’s ability to break down and digest the ingredients. The mechanical and enzymatic breakdown of a food bolus from the mouth onward produces smaller molecules. The barrier layers between the intestinal lumen and the circulation beyond the enterocyte are integral to our body’s defense mechanisms. Yet nutrient molecules will have to traverse this barrier. To easily move across this barrier, each molecule must be small enough, able to move down its concentration gradient, be soluble in an aqueous or lipid medium (depending on the barrier), and be charge compatible. For a complex and large food particle, mechanical breakdown is essential (e.g., chewing, churning) to generate the ample surface area required for exposure to enzyme action. A complex network of neural and hormonal factors regulates the function of our specialized gastrointestinal cells (epithelial, muscular, and glandular). The digestion and absorption processes are energy consuming more so for proteins than for other substances; our metabolic rate increases after a meal. Conveniently, a wide absorptive surface area is available through intestinal folding down to the villus and microvillus level. This chapter provides an overview of the basic aspects of digestion and absorption.
Carbohydrates
Dietary Forms
Carbohydrates (CHO) account for around 50% of the ingested calories in the Westernized adult diet. The forms of consumed carbohydrates change with age as an infant’s intake evolves beyond breast milk or formula. The dominant consumed dietary CHOs ( Table 2-1 ) are monosaccharides and disaccharides (mainly fructose, lactose, and sucrose) and plant starches amylose and amylopectin, which are the dominant form of plant carbohydrate storage. Consumption of high fructose corn syrup in sweet beverages has certainly increased. Our intake of animal starch (glycogen) is limited. Some carbohydrates such as cellulose cannot be broken down in the human body (see Nondigestible Carbohydrates later in this chapter).
Disaccharides | Sucrose (table sugar) | Glucose + Fructose |
Lactose | Glucose + Galactose | |
Maltose | Glucose + Glucose | |
Plant starch | Amylose | Glucose (α1,4 bonds) |
Amylopectin | Glucose (α1,4 and α1,6 bonds) | |
Animal starch | Glycogen | Glucose (α1,4 bonds) |
Nondigestible CHO | Cellulose, semicellulose, lactulose, sorbitol, sucralose | Various |
Lactose is the main CHO in breast milk and standard cow’s milk-based infant formula. For many children, cow’s milk consumption continues into adolescence and adulthood. Soy-based formulas and hypoallergenic formulas are lactose free and instead contain corn syrup (maltose and larger oligosaccharides), starch, or sucrose. Fructose imparts the sweet taste of fruits and vegetables. Fructose and glucose are the main ingredients in honey. Compared to glucose and sucrose, we perceive the fructose sweetness earlier after consumption and with more intensity. In addition, fructose can enhance the flavor and color of other food components. High fructose corn syrup differs from corn syrup in that glucose molecules are enzymatically converted to fructose in the latter, thus changing the taste and other properties. Sucrose (table sugar) is derived from cane or beet.
As infant weaning starts, the amount of consumed starch (amylopectin > amylose) increases to 50% of the total CHO intake. Amylopectin is the larger molecule of the two (molecular weight 10 9 vs 10 6 ), as its α1,6 bonds allow for branching of the polysaccharide units. Plant starch granules vary in size (e.g., potato > wheat > rice) and shape. Wheat is a unique form of starch in that the carbohydrate component is encased in a protein shell. Differences in size and packaging of the starch account for the variable degrees of digestion and absorption. The mechanical breakdown of these molecules by chewing also affects such variables, whereas food processing and preparation may alter the susceptibility of the molecular bonds within starch to enzymatic digestion.
The breakdown of CHO takes place in the gut lumen as well as at the enterocyte membrane level ( Figure 2-1 ).

Luminal Digestion
The main enzyme that digests starch is α-amylase, an endoenzyme that cleaves the α1,4 internal links in amylose and amylopectin, leaving oligosaccharides: maltose (two glucose molecules) and maltotriose (three glucose molecules). Because α-amylase does not cleave α1,6 bonds or their adjacent α1,4 bonds, digestion of amylopectin also leaves branched oligosaccharides (α-limit dextrins). Amylase activity produces only a small amount of free glucose molecules. Salivary α-amylase is secreted mainly from the parotid gland and is inactivated by gastric acid, yet some activity may linger within the food bolus itself. Amylase is also present in breast milk and plays a more significant role in premature neonates, in whom pancreatic amylase production is low ( Figure 2-2 ).

The majority of starch digestion occurs in the duodenum through the effect of pancreatic amylase. This activity is not restricted to the lumen, because amylase may adsorb to the enterocyte luminal surface. Only severe pancreatic insufficiency that leaves less than 10% normal amylase levels can affect starch breakdown.
Brush Border Digestion
Since only monosaccharides can be absorbed across the enterocyte membrane, bigger molecules such as disaccharides and the products of luminal starch breakdown need further processing. This is achieved by the hydrolases of the brush border (see Figure 2-1 ).
Maltase (glucoamylase) breaks the α1,4 links in oligosaccharides that are 5 to 9 glucose molecules long. ISOMALTASE (also called α-dextrinase) breaks α1,6 bonds, acting as a debranching enzyme. It functions in conjunction with sucrase ( Figure 2-3 ), both having their genetic coding on chromosome 3. Sucrase breaks sucrose into glucose and fructose. Sucrase-isomaltase complex cleaves its substrate by a Ping-Pong bi bi mechanism: the first enzyme reacts with one substrate to form a product and a modified enzyme, the modified enzyme then acts on the second substrate to form a final product, and then the original enzyme is regenerated.

Lactase breaks lactose into glucose and galactose; its gene is located on chromosome 2. Lactose digestion in the premature neonate may be incomplete in the small intestine but partially salvaged through colonic fermentation. In childhood, lactase levels decline from a peak at birth to less than 10% of the infant preweaning level as dietary lactose consumption falls (see Figure 2-2 ). The decline in lactase in other mammals occurs even if weaning is prolonged. In certain human populations that consume dairy products into adulthood (e.g., northern Europe), lactase activity may persist. This phenotype is inherited as an autosomal recessive trait, with intermediate activity levels in heterozygotes. Thus, the aberrant allele in the human population is considered to be the one that leads to persistence of the enzyme, not the deficiency.
Trehalase breaks down the disaccharide trehalose. Although trehalose is for most not a significant dietary component, the high concentration of trehalose in insects and fungi suggests that these have constituted a relatively large CHO source in the diet of ancient man.
Disaccharidases are synthesized in the endoplasmic reticulum of the enterocyte, modified in the Golgi apparatus, and integrated into the brush border membrane, anchored by a hydrophobic portion in their structure. Pancreatic enzymes play a role in the modification and turnover of these enzymes. The half-life of sucrase-iso-maltase drops from 20 h during fasting to 4.5 h after meals. There is generally an ample supply of disaccharidases; thus the rate of uptake of carbohydrate monomers is the limiting step for their absorption. This implies that the quantity of the ingested CHO and the rate at which it is consumed affect its absorption and potentially malabsorption. With the exception of lactase, brush border hydrolases are inducible by the presence of the substrate. Activity of mucosal carbohydrases is maximal in the duodenum and jejunum, decreasing distally along the small intestine. Most carbohydrate digestion is complete by mid-jejunum.
Transport After Digestion
Monosaccharides cross the enterocyte apical membrane via carrier-mediated transport because they are too large to allow for adequate passive diffusion. There are two families of such transporters that are responsible for the movement of monosaccharides: sodium-coupled cotransporters sodium-glucose linked transporter (SGLT) (SLC5 gene family) and the glucose transported (GLUT) (SLC2 gene family; Figure 2-4 ). SGLT1 is located in the brush border membrane, and cotransports glucose/galactose along with Na + down a sodium gradient generated by a Na + ,K + ATPase pump in the basolateral membrane. GLUT5 is also in the brush border membrane and carries fructose from the lumen into cytosol. GLUT5 allows a fructose absorption rate faster than simple diffusion down its concentration gradient. It may also be present in the basolateral membrane. GLUT2 is within the basolateral membrane and transports fructose, galactose, and glucose from the cytosol into the bloodstream. Activation of the Na + -dependent cotransport protein allows water, electrolytes, and possibly smaller digested molecules (including glucose and oligopeptides) to pass into the intercellular space through relaxation of the tight junctions. A small amount of hexoses may be utilized within the cell for metabolism.

Nondigestible Carbohydrates
Approximately 10% of ingested starch is not digested in the small intestine. Digestion-resistant starch includes complex molecules that resist amylase activity or are physically inaccessible as in intact grains. Poor chewing of large digestible molecules may compromise enzymatic exposure. Some lactose and fructose may escape complete digestion or absorption and pass to the large intestine, along with other monosaccharides such as lactulose, sorbitol, and sucralose, which cannot be digested or absorbed in the human intestine. Cellulose and hemicellulose are present in the fruit and vegetable structure. Cellulose is a polymer of glucose molecules linked by β1,4 bonds that, unlike α1,4 bonds, resist digestion by α-amylase. Hemicellulose is a polymer of pentose and hexose molecules in straight and chained forms. Resistant starches constitute dietary “fiber” together with nondigestible noncarbohydrate components present in the plant cell wall (e.g., phytates, lignins). Nondigestible carbohydrates are fermented by colonic bacteria, leaving short-chain fatty acids that are readily absorbed and may account for a minute caloric source in the healthy state, in addition to possibly having cellular trophic properties. By-products of this process are lactic, acetic, propionic, and butyric acids with methane and hydrogen accounting for flatus. Although excessive consumption of nondigestible carbohydrates can result in undesirable gastrointestinal symptoms, dietary fiber may offer multiple health benefits.
Proteins
Protein Sources
To reduce the consumption of amino acids for energy production, the intake of proteins must be accompanied by other calorie sources. In addition to dietary protein, the gastrointestinal tract recycles endogenous proteins in digestive juices and sheds epithelial cells, amounting to as much as 65 g daily in adults. The quality of dietary protein relates to its content of essential amino acids (valine, leucine, isoleucine, phenylalanine, lysine, tyrosine, methionine, tryptophan, and histidine) that cannot be synthesized in humans. An egg, for example, has a high-protein biologic value because it is rich in essential amino acids. Plant proteins are less digestible than animal proteins and contain fewer essential amino acids. A cooked protein will have a simpler structure due to denaturation, which can be achieved through heat, mechanical pressure (pounding), or exposure to acid and sodium chloride. Co-ingesting the protein with reducing sugars such as fructose can alter its molecular structure and affect digestibility. Proteins with high proline content (e.g., casein, gluten, collagen, and keratin) are incompletely digested by pancreatic proteases. Other proteins that escape digestion include secretory immunoglobulin A (IgA) and intrinsic factor.
Luminal Digestion
Gastric Phase
Much of our earliest knowledge of the gastric phase comes from the work of William Beaumont in the 1820s. Beaumont was a surgeon who performed different experiments by placing and retrieving food items through a gastrostomy that a patient was left with after a gun wound. He observed that “those articles of diet which were submitted to the actions of the gastric juice, either artificially when out of the stomach or in the stomach by natural process, were dissolved in proportion to the fineness of their division or their solidity, the one rapidly and the other slowly.” This emphasizes the importance of the mechanical breakdown of food to facilitate digestion and the role of cooking in softening foods. Gastric hydrochloric acid denatures protein and activates pepsinogen to pepsin. Pepsinogen is secreted by chief cells and acts as an endopeptidase, breaking peptide bonds within the polypeptide and leaving shorter polypeptides with a small number of free amino acids. Three pepsin isoenzymes have been identified, all optimally active at a pH range of 1 to 3. The duodenal alkaline medium irreversibly inactivates pepsin. Both pepsin and gastric acid production and secretion are stimulated by gastrin, acetylcholine, and histamine. The gastric phase does not seem critical in protein breakdown, because patients with decreased acid output and/or gastrectomy do not necessarily lose protein.
Intestinal Phase
The main protein digestion site is the proximal small intestine upon exposure to the pancreatic fluid. Unlike amylase and lipase, pancreatic proteases are secreted as proenzymes. The presence of food in the duodenum stimulates contractions of the gallbladder and subsequent bile flow, along with stimulating secretion of pancreatic fluid. Although mediators of pancreatic stimulation are incompletely understood, the cholinergic intestinal system appears to have greater influence than cholecystokinin for pancreozymes, whereas secretin mainly promotes pancreatic bicarbonate flow. Bicarbonate provides an alkaline pH of greater than 5, which is required for optimal enzyme function. An over-acidic environment, as seen in Zollinger-Ellison syndrome, deactivates pancreatic enzymes. In response to the presence of bile acids and trypsinogen, enterokinase (enteropeptidase) is released from the brush border cells. Trypsinogen, the only substrate of enterokinase, is the most abundant proenzyme in pancreatic juices. The subsequent removal of a hexapeptide from the N terminus of trypsinogen yields the active form, trypsin, which activates the other enzyme precursors as well as its own ( Figure 2-5 ). Pancreatic proteases are either endopeptidases or exopeptidases, depending on the site of the peptide bonds each acts upon ( Table 2-2 ). Endopeptidases cleave peptide bonds within the polypeptide chain, whereas exopeptidases remove a single amino acid from the carboxyl terminal. About 30% to 40% of the products of this process are amino acids, and 60% to 70% are oligopeptides up to six peptides long. Endogenous proteins (including enzymes) are digested and processed in a manner to that of exogenous proteins.

Enzyme | Protein Substrate |
---|---|
Endopeptidases | |
Trypsin | Basic amino acids (lysine, arginine); pancreatic proenzymes |
Chymotrypsin | Aromatic amino acids (glutamine, leucine, methionine) |
Elastase | Aliphatic (nonpolar) amino acids |
Exopeptidases | |
Carboxypeptidase A | Aromatic, aliphatic amino acids |
Carboxypeptidase B | Basic amino acids |
Pancreatic enzymes also release cobalamin (vitamin B 12 ) from the R protein, allowing the former to bind to intrinsic factor (see Vitamin B 12 ). The enzymes may also play a role in gut immunity against microbials and interact in the modification-regulation of various brush border enzymes such as disaccharidases. Exposure to trypsin changes procolipase to colipase, a key player in the assimilation of fat.
Brush Border and Intracellular Digestion
Brush Border
Unlike carbohydrates, where only monosaccharide units are transported across the enterocyte membrane, small peptides can move directly from the lumen into the enterocyte ( Figure 2-6 ). It has been shown in animals with pancreatic insufficiency secondary to pancreatic duct ligation that nearly 40% of ingested proteins were absorbed. In fact, the transport of small peptides may be more efficient than that for amino acids. Because almost all protein that enters the portal vein is in the form of amino acids, further digestion of any oligopeptides (which have 2 to 6 amino acids) must take place either at the brush border level or within the enterocyte cytoplasm.

An oligopeptide’s length determines the rate and the site (brush border versus intracellular) of its assimilation. The brush border peptidases are active at neutral pH and include an array of aminopeptidases, carboxypeptidases, endopeptidases, and dipeptidases. These enzymes possess a combined ability to digest hexapeptides or smaller chains into amino acids, dipeptides, and tripeptides that are actively transported across the luminal enterocyte membrane. In contrast to disaccharidases, synthesis of the brush border peptidases occurs in the rough endoplasmic reticulum, with little posttranslational enzyme modification within the cell or by pancreatic enzymes at the brush border. Mucosal enzymes also include folate conjugase, which hydrolyzes ingested folate, and angiotensin-converting enzyme.
Cytoplasm
Cytosol peptide hydrolases differ in structure and electrophoretic mobility from those in the brush borders and are predominantly dipeptidases and tripeptidases. Further assimilation of small polypeptides into free amino acids takes place in the cytoplasm; however, the capacity to digest peptides more than three amino acids long is lacking. Iminodipeptidase (also called prolidase) is an intracellular hydrolase with specificity to proline-containing dipeptides, which resist luminal digestion but pass into the cytoplasm. In contrast to the brush border enzymes, cytosol peptidases are not exclusive to the intestine and are present in other body tissues.
Transport After Digestion
Amino Acids
The complex process of transmembrane movement remains incompletely understood. Dipeptides and tripeptides do not compete with amino acids for transport (see Figure 2-6 ). Amino acid transport proteins are numerous and group specific for neutral, basic, and acidic amino acids, with some overlap. A few transport proteins have been extensively studied and characterized. Absorption is maximal in the proximal intestine and occurs by active diffusion, Na + -cotransport, and to a lesser extent, simple and facilitated diffusion. The rate of absorption varies for different amino acid groups, being highest for branched-chain amino acids. Vasointestinal polypeptide and somatostatin slow these processes. As noted in glucose transport, activating the cotransport protein may allow paracellular movement of intestinal contents.
Dipeptides and Tripeptides
In contrast to amino acids, dipeptides and tripeptides are carried by a single membrane transporter with a broad substrate specificity. This transporter utilizes an H + gradient and is uniform along the small intestines. The human peptide transporter has been successfully cloned. A brush border Na + , H + exchange pump, along with Na + , K + ATPase in the basolateral membrane, maintains this confined acidic milieu ( Figure 2-7 ). The ability of dipeptides and tripeptides to be carried into the enterocyte contributes to the lack of specific amino acid deficiency in hereditary disorders of amino acid transport, as seen in Hartnup disease and cystinuria. Both substrates of these carriers are absorbed normally in disease states if presented in the form of small peptides. In the neonatal period, uptake of whole polypeptide macromolecules occurs possibly by pinocytosis or receptor-mediated endocytosis, allowing for passage of such molecules as immunoglobulins in the first 3 months of life.

Exit From the Enterocyte
The movement of amino acids across the basolateral membrane occurs by facilitated and active transport. This is handled by transport proteins different from those in the brush border membrane. In addition to exporting amino acids into the portal circulation, these amino acid transport proteins take up amino acids into the enterocyte for use during fasting periods. The basolateral membrane also possesses a dipeptide and tripeptide transport system similar to the one in the brush border membrane, allowing a small amount of intact small peptides to enter the bloodstream.
About 10% of the amino acids absorbed into the mucosa are used for the enterocyte’s own protein synthesis in vitro. Luminal protein sources are more readily used than systemic protein, especially in apical villous cells. It has been shown in animals that exclusive parenteral nutrition can lead to mucosal atrophy.
Lipids
Dietary Forms
Up to 90% of fat in the average human diet consists of triglycerides; the remainder is phospholipids, plant and animal sterols (including cholesterol), and fat-soluble vitamins. In a triglyceride, a backbone of glycerol carries three fatty acids of variable lengths. Animal-derived triglycerides generally have long-chain saturated fatty acids (>14 carbon units), the majority being oleate and palmitate. Plant fatty acids are polyunsaturated and include linoleic and linolenic acids, which cannot be synthesized de novo in humans and are therefore essential. Medium-chain triglycerides have fatty acids with 8 to 12 carbons. Processing of vegetable fat involves hydrogenation, which increases the melting point, saturates the covalent bonds within the fatty acid, and changes double bonds from cis to trans isomers. A phospholipid is composed of a backbone of lysophosphatidylcholine and one fatty acid. The average adult diet contains 1 to 2 g of phospholipids, while 10 to 20 g is secreted daily in bile. Phospholipids are also recycled from cell membranes of shed enterocytes. The main dietary phospholipid is phosphatidylcholine (lecithin), and the predominant fatty acids in phospholipids are linoleate and arachidonate. Cholesterol, in animal fat, is the main dietary sterol in the Westernized diet. Fat-soluble vitamins are discussed later in this chapter.
Lipids are divided into polar and nonpolar, depending on the nature of their interactions with water. Triglycerides are insoluble in water and form an unstable layer, whereas polar phospholipids can shape into a more stable form. This is key to understanding the dynamics of lipid digestion and absorption across the water phase in the intestinal lumen, the epithelial membrane lipid phase, and later the lymphatic and blood-water phase. To provide a better exposed, more stable enzyme substrate, ingested lipids are mechanically and enzymatically broken down to smaller units, and then appropriately coated with such hydrophilic molecules such as phospholipids and bile salts to help cross through different aqueous phases.
Luminal Digestion
See ( Figure 2-8 ).

Gastric Phase
The digestion of triglycerides begins in the stomach, where lingual and gastric lipases are active in the acid milieu. The degree of relative activity of each varies among different species. Lingual lipase is secreted from Ebner’s glands, which are located on the posterior tongue. Both enzymes break down short- and medium-chain triglycerides more efficiently than long chain ones and cannot process phospholipids or sterols. In neonates, pancreatic production of lipase is not fully developed (see Figure 2-2 ). Breast milk is rich in medium- and short-chain fatty acids that are adequately handled by breast milk-derived lipase (carboxyl ester lipase) and infantile gastric lipase. In adults, it is estimated that 10% to 30% of ingested lipids are digested before the duodenal stage, yielding diacylglycerols and free fatty acids. Gastric lipase has high activity in patients with cystic fibrosis in the presence of reduced pancreatic lipase and lower pH affecting its activity. There is no absorption of fat in the stomach, except for short-chain fatty acids. Nevertheless, the stomach is the major site of fat emulsification, the mechanical fragmenting of larger lipid globules into smaller, uniformly distributed particles ( Figure 2-9 ). Breast milk fat emulsion droplets are relatively small. In addition, gastric lipase releases some fatty acids, which together with the ingested dietary phospholipids offer a hydrophilic “coat” around the intact triglycerides to provide a more stable suspension of emulsified fat droplets. The coordinated gastric propulsion-retropulsion contractions leave lipid droplets smaller than 0.5 µm, which are then squirted through the pylorus.

Small Intestinal Phase
After meal ingestion, vagal stimulation and cholecystokinin (CCK) stimulate gallbladder contractions and relaxation of the sphincter of Oddi, thereby allowing bile flow into the duodenum. The three main bile acids are cholic, deoxycholic, and chenodeoxycholic acids. Bile acids are secreted almost exclusively in conjugated form, predominantly to glycine and less so taurine. Such modification enhances the water solubility of bile acids, even in slightly acidic medium, by lowering the critical micellar concentration. Conjugation also confers some resistance to pancreatic digestion and prevents calcium-bile salt precipitation. In addition to bile acids, bile is rich in phospholipids; both compounds are amphipathic, having both hydrophilic and lipophilic portions. The concentration of bile acids is usually well above a critical level where micelles (water-soluble aggregates) are formed upon mixing with digested lipids. Micelles are 100 to 500 times smaller in diameter than emulsion particles, which explains why a meal containing fat would look like a water-clear micellar solution in the proximal small intestine. The orientation within a micellar structure is such that the hydrophobic bile acid parts cover the insoluble molecules within, whereas the hydrophilic portion lines the outer layer, allowing stability in the luminal aqueous phase. However, as a result, the hydrophobic portion of the lipid where lipase acts is contained deep within the emulsion droplet. To allow exposure to lipase, pancreatic phospholipase A 2 is activated by bile acids and calcium to break the phospholipid coat, leaving fatty acids and lysophosphatidylcholine units. The optimal action of phospholipase A 2 requires a bile salt-to-phosphatidylcholine molar ratio of 2:1. It has been shown that the presence of bile acids inactivates lipase, which led to the discovery of its cofactor, colipase, in 1963. Colipase is secreted from the pancreas as procolipase at a 1:1 ratio to lipase, which it carries in proximity to the triglyceride. A by-product of the activation of procolipase by trypsin is a pentapeptide, enterostatin, which is believed to play a role in satiety after fat ingestion. The products of lipase’s activity are 2-monoacylglycerols and free fatty acids. Most ingested cholesterol is in the free sterol form, and a small amount is in cholesterol-ester form, which requires digestion by cholesterol esterase, also called nonspecific lipase. The luminal end products of lipid digestion (fatty acids, 2-monoglycerides, glycerol, lysophosphatidylcholine, and free cholesterol) are insoluble in water except for short- and medium-chain fatty acids and glycerol, which are sufficiently soluble to pass through the unstirred water layer that lines the intestinal epithelium.
Enterohepatic Bile Circulation
Liver cells synthesize and conjugate bile acids starting from cholesterol. Conjugated bile acids are reabsorbed through the enterohepatic circulation. Both processes are in balance to keep an adequate bile acid pool. Because conjugated bile acids are in ionized form in the alkaline intestinal milieu, they cannot be absorbed passively across the enterocyte membrane. It has been shown that active transport of these bile acids takes place in the distal ileum. Ileal bile acid absorption involves Na + cotransport down a concentration gradient secured by the basolateral membrane Na + ,K + ATPase ( Figure 2-10 ). Within the enterocyte, bile acids are carried by binding proteins that protect the cell against injury from the otherwise free acids. Bacterial enzymatic action in the distal small and large intestines leads to deconjugation of bile acids that escape ileal absorption and removal of the 7-hydroxy group, leaving deoxy bile acid forms. A fraction of the unconjugated bile acids are readily absorbed into the gut epithelium, given their lipophilic properties. The acidic environment in the colon results in the change of bile acids to solid form. Only a small amount of bile acids is lost in feces. (See also Chapter 3 .)
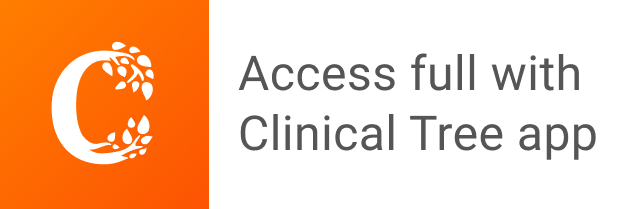