Fig. 1
Segments of the liver (Reprinted from Clin Liver Dis, 4, Ghobrial RM, Amersi F, Busuttil RW, Surgical advances in liver transplantation. Living related and split donors, 553–565, Copyright (2000), with permission from Elsevier)
The liver has a unique dual blood supply: arterial supply from the hepatic artery, a branch of the celiac axis, and venous supply from the portal vein formed by the union of the splenic and superior mesenteric vein. Despite liver mass constituting only 2.5 % of the total body weight, the total hepatic blood flow is approximately 100 mL/100 g/min, or 25 % of cardiac output. The hepatic artery supplies approximately 25–30 % of hepatic blood flow and 45–50 % of the oxygen requirement, while the portal vein supplies 70–75 % of hepatic blood flow and 50–55 % of oxygen. The venous drainage is through the right, middle, and left hepatic veins, which merge into the IVC. The valveless portal vein is a low pressure/low resistance circuit, while the hepatic artery is a high pressure/high resistance system. Hepatic blood flow is primarily regulated by local metabolic demand with an inverse relationship between portal venous and hepatic arterial flow: an increase in the hepatic adenosine level triggered by a reduced portal venous flow increases hepatic arterial blood flow (Gelman and Ernst 1977; Lautt et al. 1985). The hepatic artery buffer response appears to be functional even after liver transplantation (Payen et al. 1990), and this response may be responsible for the development of the small-for-size syndrome after living donor or split liver transplantation (Kiuchi et al. 1999). Small-for-size syndrome develops in a patient who received a donor graft that was less than 1 % of the recipient’s body weight and is caused by decreased hepatic arterial flow in response to increased portal venous flow and pressure. Subsequently, a prolonged postoperative reduction in hepatic arterial flow can lead to centrilobular tissue necrosis, biliary ischemia, and hepatic arterial thrombosis (Smyrniotis et al. 2002). There is no buffer response in the portal system because the portal vein cannot regulate its blood flow. Therefore, alterations in the hepatic arterial blood flow do not induce compensatory changes in the portal blood flow (Lautt 1983).
The mean pressure in the hepatic artery is similar to that in the aorta, while portal vein pressure ranges between 6 and 10 mmHg. The portal pressure depends primarily on the degree of constriction or dilatation of the splanchnic arterioles and on intrahepatic resistance. Both afferent systems merge at the sinusoidal bed, where the pressure is estimated to be 2–4 mmHg higher than that in the IVC. The liver serves as a blood reservoir, and it replenishes blood volume of up to 25 % rapidly in the case of an acute bleeding episode (Lautt 2007). Hepatic blood volume may expand considerably in cardiac failure by venous congestion.
The liver is innervated by the left and right vagi, the right phrenic nerve, and fibers from the T7–T10 sympathetic ganglia. The hepatic artery is innervated mainly by sympathetic fibers, and hepatocytes, by the unmyelinated sympathetic fibers. The bile ducts are innervated by both sympathetic and parasympathetic fibers. The role of hepatic innervation is unclear, as denervation of the transplanted liver does not affect its function (Kjaer et al. 1994).
Bile flow begins from the bile canaliculi to the common bile duct. Hepatic lymph forms in the space between the sinusoid and the hepatocyte (space of Disse) and flows to lymph nodes in the hilum and IVC. The transdiaphragmatic lymphatic flow is the cause of pleural effusions in the presence of large ascites.
The liver is made of parenchymal cells (hepatocytes) and non-parenchymal cells (sinusoidal endothelial, Kupffer, stellate, dendritic, and lymphocyte). Hepatocytes make up 60–80 % of liver cells and carry out hepatic metabolic, synthetic, and detoxification functions. Polyhedral hepatocytes are arranged in one-cell thick plates with endothelium-lined sinusoids on both sides. Each hepatocyte cell membrane has three distinct membrane domains. The sinusoidal membrane is adjacent to the sinusoidal endothelium and has numerous microvilli abutting into the space of Disse. Fenestrae within the sinusoidal endothelium without the basement membrane permit intimate contact between sinusoidal blood and the hepatocytes to allow the passage of big molecules, including lipoproteins. Liver sinusoidal endothelial cells make up 15–20 % of liver cells and release nitric oxide to regulate vascular resistance. They are, along with dendritic cells and lymphocytes, part of the innate immune system. The space of Disse contains phagocytic Kupffer cells that participate in the hepatic inflammatory process. The Ito cells, also known as stellate cells, are the major site of vitamin A storage, and their activation results in hepatic fibrosis and cirrhosis. Reticulin fibers in the space of Disse support the sinusoidal framework, and weakening of these supporting fibers results in rupture of sinusoidal walls and formation of blood-filled cysts known as peliosis hepatis, a forerunner of cirrhosis. The apical membrane circumscribes the canaliculus, the earliest component of the biliary system. The lateral hepatic membrane is found between adjacent hepatocytes.
The functional unit of the liver is the acinus. Terminal portal veins communicate with terminal hepatic venules, with sinusoids bridging the gap between the two vessels (Fig. 2). Each sinus contains three zones with equal blood pressure and oxygen content. The periportal zone (Zone 1) receives blood highest in oxygen content and the pericentral or perivenular zone (Zone 3) receives blood lowest in oxygen content. As a result, the hepatocytes in the perivenular zone (Zone 3) are more vulnerable to ischemic damage and nutrient depletion. Oxidative and reductive functions are predominantly performed by hepatocytes at the periportal zone and glucuronidation is performed by those at the perivenular zone, although hepatocytes of the two different zones are functionally integrated (Lamers et al. 1989). The unique structure of the liver acinus is well-suited for bidirectional transfer of nutrients. The low pressure in the portal venous system allows blood to flow slowly through the sinusoids. Hepatic arterial blood flows mainly to the terminal bile canaliculi, although it augments sinusoidal flow to give a gentle pulsatility. In patients with liver cirrhosis, the sinusoids acquire features of systemic capillaries: the space of Disse widens with collagen deposits at the basement membrane, endothelial fenestrations become smaller and fewer, and hepatic microvilli efface. All of these changes reduce transport across the sinusoidal walls and result in hepatic dysfunction. Furthermore, widespread fibrosis and scarring reduce the number and size of the small portal and hepatic veins and increase intrahepatic vascular resistance to the development of portal hypertension (Popper 1977). The sluggish blood flow in the altered vascular architecture promotes thrombosis, causing further cell necrosis and fibrosis (Wanless et al. 1995).


Fig. 2
Schematic diagram of the acinus
The liver undergoes rapid regeneration through proliferation of hepatocytes to maintain the critical mass necessary for normal liver function. For example, the newly transplanted hemiliver from a living related donor regenerates to about 85 % of its original whole liver size in 7–14 days. The major hepatic growth factors are epidermal growth factor and hepatocyte growth factor (Michalopoulos 1990). Administration of the epidermal or hepatocyte growth factor to normal rats, however, does not cause hepatocyte replication. This negative response suggests that liver regeneration involves a two-step process: the initial signal generated by an acute increase in metabolic demand associated with the loss of hepatocytes triggers a set of early response genes that prime hepatocytes to respond to various growth factors. In apoptosis or programmed cell death, aging hepatocytes are removed and new cells are produced in a continuous manner (Ellis et al. 1991).
Hepatic Function
The liver has three major functions: metabolism, bile production and secretion, and filtration of harmful substances.
Carbohydrate Metabolism
The principal role of the liver is to provide the body with normal glucose levels, which are regulated by insulin, glucagon, growth hormone, and catecholamines (Pilkis and Granner 1992). The liver converts glucose into glycogen (glycogenesis) and utilizes glucose for the synthesis of fatty acids.
Cirrhotic patients are frequently hyperglycemic although their insulin level is elevated (Petrides and DeFronzo 1989). This insulin resistance is caused by multiple mechanisms. Cirrhotic patients have an increased basal metabolic rate and use preferentially fatty acids as an energy source. Reduced glucose uptake and limited glucose storage in the liver and muscle lead to hyperglycemia. Other contributing factors are increased serum fatty acids, which inhibit glucose uptake by muscle; altered second messenger activity after insulin binding to its receptors; an increased concentration of serum cytokines associated with elevated levels of endotoxins; and increased levels of glucagon and catecholamines.
Protein and Amino Acid Metabolism
The liver is the major organ for protein synthesis, and albumin is the most important protein product. Albumin is the major contributor to plasma oncotic pressure and binds and transports bilirubin, hormones, fatty acids, and other substances. Hypoalbuminemia is commonly caused by decreased hepatic synthetic function, although it can be secondary to an enlarged volume of distribution, reduced level of amino acid precursors, and losses into the urine, peritoneum and pleural cavity, and leads to peripheral edema, ascites, and pleural effusions. The low serum oncotic pressure stimulates the hepatic albumin synthesis in healthy subjects, but this is impaired in patients with cirrhosis (Pierrangelo et al. 1992). The liver synthesizes all coagulation factors (except von Willebrand factor) and protein C and S. Factors II, VII, IX, and X undergo a posttranslational vitamin K-dependent modification involving γ-carboxylation of specific glutamic acid residues in the liver.
The liver is the primary site of interconversion of amino acids. Anabolic processes synthesize proteins from amino acids, while catabolic processes convert amino acids either to keto acids by transamination or ammonia by oxidative deamination. Ammonia, in turn, is converted to urea by the Krebs-Henseleit cycle. In patients with liver disease, derangement of both anabolic and catabolic processes results in decreased production of blood urea nitrogen (BUN) and accumulation of ammonia, a contributing factor in the development of hepatic encephalopathy. The liver produces acute-phase reactants, such as α-fetoprotein, ceruloplasmin, fibrinogen, transferrin, complement, and ferritin. They are expressed during acute and chronic systemic inflammation, and their activation is mediated by interleukin-6, tumor necrosis factor, interferon- γ, and glucocorticoids.
Lipid Metabolism
The liver takes up fatty acids and cholesterol from diet and peripheral tissues to produce and release lipoprotein complexes into circulation. Fatty acids released from adipocytes are bound to serum albumin and transported to the liver for the synthesis of phospholipids and triglycerides. The liver produces fatty acids from small molecular weight precursors, and cholesterol synthesis is regulated by the rate-limiting enzyme 3-hydroxyl-3 methylglutaryl coenzyme A reductase (HMG-CoA reductase). Lipids are exported out of the liver by very low-density lipoprotein (VLDL) particles, which are the major carriers of plasma triglycerides during non-absorptive states. Lipids are temporarily stored in the liver as fat droplets, or as cholesteryl esters in the case of cholesterol, and are directly excreted into bile or metabolized into bile acids. The liver is the major site for sterol excretion and production of bile acids.
Various abnormalities in lipid metabolism are common in liver disease. Hypertriglyceridemia (250–500 mg/dL) is the most common presentation and may be caused by decreased synthesis of lipoproteins, decreased hepatic clearance of lipoprotein complexes, or re-entry of biliary content into the serum. Alcoholic liver injury results in increased fatty acid synthesis and steatosis (Lieber 1993). Paradoxically, an increased high-density lipoprotein (HDL) 3 level has been noted with moderate alcohol consumption, which may explain the reduced risk of atherosclerosis in these patients (Chait and Brunzell 1990). Patients with cholestatic liver diseases have elevated total serum cholesterol and triglycerides because the bile is rich in cholesterol, phospholipids, and lecithin.
Detoxification and Hormone Alteration
The liver eliminates drugs through two types of reactions. The phase 1 reactions include oxidation, reduction, hydroxylation, sulfoxidation, deamination, dealkylation, and methylation of reactive substances. These reactions involve systems such as cytochrome P450 and typically occur in the periportal area of the liver. The phase 2 reactions, which transform lipophilic agents into more water-soluble compounds, take place in the pericentral area. In patients with liver disease, hepatic drug clearance is usually reduced due to the enlarged volume of distribution and decreased hepatic metabolism. As a result, a large initial dose of medications followed by small, titrated maintenance doses are required to achieve the desired pharmacologic effects.
Several hormones are deactivated or altered in the liver. The deactivated hormones are insulin, glucagon, steroid hormones, aldosterone, thyroxine, and triiodothyronine. The liver converts testosterone into androsterone and estrogen into estrone and estriol. Abnormal levels of estrogen and testosterone in patients with liver disease lead to testicular atrophy, loss of pubic and axillary hair, spider angioma, and gynecomastia.
Excretory Function
The liver removes various substances from the body, and bile formation is one of the most important excretory functions. When membranes of old erythrocytes rupture, the released hemoglobin is taken up by the reticuloendothelial cells and is split into heme and globin. Heme converts to biliverdin, which, in turn, is reduced to free bilirubin and released into the plasma. The free bilirubin–albumin complex is taken up by the hepatocytes. Bilirubin conjugates primarily with glucuronic acid and is actively transported into the bile. A small portion of conjugated bilirubin returns to the plasma directly from the sinusoids or indirectly by absorption from the bile ducts and lymphatics. Bilirubin is converted into urobilinogen by the intestinal bacterial flora. Some urobilinogen is reabsorbed through the intestinal mucosa and is re-excreted into the intestine. Bile acids, which enhance absorption of vitamin K, are also excreted into the bile by the liver.
Filtration Function
The liver, located between the splanchnic and systemic venous system, acts as a vascular filter. Kupffer cells phagocytose immune complexes, endotoxins, and bacteria in the portal venous blood and process antigens for presentation to immunocompetent cells. The liver also removes activated coagulation elements from circulation to prevent excessive coagulation or fibrinolysis.
Pathophysiology of Liver Cirrhosis
Liver cirrhosis is defined as progressive fibrosis and the formation of regenerative nodules, and is the final common pathway in which hepatocytes are replaced by connective tissue after various, repetitive insults. The amount of remaining functional hepatic mass and the degree of architectural distortion determine the functional state of the liver. Portal hypertension is inevitable in advanced cirrhosis and leads to ascites, variceal bleeding, and encephalopathy. The severity of cirrhosis is frequently classified using the Child-Pugh score (Table 1), and a score of >6 suggests a short life expectancy.
Table 1
Child-Pugh score
Presentation | Points | ||
---|---|---|---|
1 | 2 | 3 | |
Albumin (g/dL) | >3.5 | 2.8–3.5 | <2.8 |
Prothrombin time | |||
Seconds prolonged | <4 | 4–6 | >6 |
INR | <1.7 | 1.7–2.3 | >2.3 |
Bilirubin (mg/dL) | |||
Hepatocellular disease | <2 | 2–3 | >3 |
Cholestatic disease | <4 | 4–10 | >10 |
Ascites | Absent | Mild–moderate | Tense |
Encephalopathy | None | Grade 1–2 | Grade 3–4 |
Central Nervous System
Hepatic encephalopathy is a reversible neuropsychiatric condition in both acute and chronic liver failure. In chronic liver disease, hepatic encephalopathy develops in 28 % of patients within 10 years of compensated cirrhosis and is associated with spontaneously developed or surgically created portosystemic shunting (Butterworth 2001). The degree of encephalopathy is stratified by a coma scale: Grade 1, subtle confusion; Grade 2, somnolence; Grade 3, unconsciousness with response to pain stimulation; and Grade 4, deep coma. Clinically, asterixis, flapping tremor, and fetor hepaticus (musty, sweet breath odor) are confirmatory of hepatic encephalopathy.
The main cause of hepatic encephalopathy is the altered expression of several genes for various neurotransmitter proteins in the brain (Butterworth 2001). Decreased expression of the glutamate transporter (GLT-1) increases extracellular brain glutamate. An increased expression occurs in some receptors: monoamine oxidase increases degradation of monoamine transmitters, the peripheral-type benzodiazepine receptor increases inhibitory neurosteroids, and neuronal nitric oxide synthase increases nitric oxide production. Although its plasma level is not closely related to the severity of encephalopathy, ammonia is still considered to be a major contributing factor. Ammonia and manganese are known to alter the expression of the peripheral-type benzodiazepine receptor and neuronal nitric oxide synthase in exposed cells (Warskulat et al. 2001).
Magnetic resonance spectroscopy reveals brain edema and increased brain glutamine/glutamate in the frontal and parietal lobes; histologic findings are swelling and glycogen deposition in astrocytes. These changes in the brain coincide with impairment in the visuopractic capacity, visual scanning, and perceptual–motor speed on neuropsychiatric testing (Tarter et al. 1984). Subclinical hepatic encephalopathy can be detected by having patients perform a simple timed connect-the-numbers test.
Treatment of hepatic encephalopathy is based on the ammonia-lowering strategy, such as protein restriction, oral non-absorbable antibiotics, and lactulose. Rifaximin reduces the plasma ammonia level by destroying intestinal bacteria that produce urease. Metronidazole (800 mg/day) is another antibiotic, although its adverse effects limit its use to 1 week at a time. Lactulose is a substrate for gut bacteria and reduces the formation of ammonia by lowering intestinal pH. Rifaximin and lactulose are commonly used together, and the antibiotic is discontinued once eradication of disaccharide-metabolizing intestinal bacteria is indicated by an increase in stool pH. Oral or parenteral ornithine aspartate, a substrate for the conversion of ammonia to urea and glutamine, has effects similar to those of lactulose, but with fewer adverse effects. In patients with severe encephalopathy, the molecular-absorbent recycling system (MARS) may be utilized to remove small and middle molecular weight water-soluble substances (Sorkine et al. 2001). The system appears to increase blood pressure and systemic vascular resistance, possibly by removing nitric oxide.
In fulminant hepatic failure, progressive hepatic coma is accompanied by a gradual increase in cerebral blood flow and intracranial pressure (ICP) (see Chap. 12, “Fulminant Hepatic Failure: Diagnosis and Management”). Subsequently, vasogenic cerebral edema and severe intracranial hypertension develop and approximately 30–50 % of patients die of brain herniation. Monitoring of ICP using a Ladd epidural sensor is useful in detecting intracranial hypertension, monitoring the therapeutic effects, and identifying patients who would survive after transplantation without neurologic damage (Lidorsky et al. 1992). Non-invasive neurologic assessment includes transcranial Doppler (TCD) to measure cerebral bloodflow velocity, determination of the cerebral metabolic rate for oxygen by calculating the oxygen content difference between arterial and jugular bulb venous blood, evoked potentials, and serial computed tomography (CT) scans (Aggarwal et al. 1994). Treatment includes osmotic and loop diuretics, barbiturate-induced coma, and hypothermia. The definitive treatment is usually transplantation.
Cardiovascular System
The presence of hyperdynamic circulation with a markedly increased cardiac output and decreased systemic vascular resistance was first described by Kowalski and Abelmann in the early 1950s (Kowalski and Abelmann 1953). Several hypotheses have been proposed to explain this phenomenon, including an overactive sympathetic nervous system, inadequate clearance of vasoactive substances by the diseased liver, the presence of arteriovenous shunts, nitric oxide-induced vasodilation, and relative hypoxia in peripheral tissues (Benoit et al. 1984; Yokoyama et al. 1989; Kalb et al. 1993; D’Souza et al. 1993).
Although cardiac output is frequently two to three times normal, impaired systolic and diastolic function together with attenuated cardiac responsiveness to stimuli suggests that cardiomyopathy is present in cirrhotics (cirrhotic cardiomyopathy) (Lee 1989). Caramelo et al. noted a 50 % decrease in cardiac output with volume expansion in a CCl4-induced cirrhotic rat model (Caramelo et al. 1986). In another rat model, the chronotropic response to isoproterenol was attenuated compared with that in control animals (Lee et al. 1990). Cardiac response to physical exercise is blunted in patients with cirrhosis, indicated by alterations in the pre-ejection period, isometric contraction time, and ratio of the pre-ejection period to left ventricular ejection time. In addition, abnormalities in myocardial diastolic indices suggest non-compliant ventricles. Histologically, myocardial fibrosis, mild subendocardial edema, and vacuolation of myocyte nucleus and cytoplasms are observed. The development of cirrhotic cardiomyopathy is multifactorial. It appears that the β-receptor system, the main stimulant of the ventricle, is dysfunctional. In humans, lymphocyte β-receptor density, which reflects cardiac β-receptor status, is reduced in patients with severe ascites (Gerbes et al. 1986), and β-receptor density of the cardiomyocyte sarcolemmal plasma membrane is reduced in cirrhotic rats (Liu and Lee 1999). Further, the β-receptor signal transduction pathway is impaired at several levels (Ma et al. 1996). Although cardiac contractile impairment may result from overactivity of the muscarinic M2 receptor, the receptor density and binding affinity are unchanged, suggesting normal parasympathetic function (Jaue et al. 1997). High serum catecholamine levels, a result of desensitization and down-regulation of β-receptors, may lead to myocardial dysfunction in the presence of α-mediated coronary vasoconstriction. Additionally, overproduction of nitric oxide inhibits β-receptor-stimulated cyclic adenosine monophosphate (cAMP) release, causing myocardial dysfunction and vasodilation (Hare and Colucci 1995).
Coronary artery disease (CAD) was previously believed to be relatively uncommon in patients with cirrhosis as a result of generalized vasodilation and elevated levels of HDL and estrogen. In addition, autopsy findings showed relatively fewer atherosclerotic changes and myocardial infarction. However, studies have shown that CAD is not uncommon, and moderate-to-severe CAD was found in approximately 27 % of patients who underwent coronary artery catheterization as a part of liver transplantation workup (Carey et al. 1995). In another study of 161 liver transplantation candidates who were at risk for CAD and referred for coronary angiography, 25 % of patients had at least one moderate or severe (>50 %) coronary stenosis (Tiukinhoy-Laing et al. 2006).
Endocarditis is three times more common in patients with liver disease (Snyder et al. 1977). This is attributed to translocation of intestinal bacteria through the intestinal wall and portosystemic collaterals, and reduced immune response. The incidence of pericardial effusion in cirrhotic patients is approximately 32–63 % and correlates with the degree of liver failure (Shah and Variyam 1988). The effusion is usually small and may require drainage if it affects cardiac function. Patients with liver disease exhibit three common cardiac electrophysiological disturbances: electromechanical dissociation, prolongation of ventricular repolarization (the Q–T interval), and chronotropic incompetence (Milani et al. 2007).
Pulmonary hypertension associated with portal hypertension was first described in 1951 (Mantz and Craige 1951). Pulmonary hypertension defined as a mean pulmonary artery pressure of >25 mmHg and pulmonary vascular resistance of >240 dyn/s/cm−5 (3 Wood units) is more common in patients with liver disease, with a prevalence of 0.25–0.73 % (Lebrec and Capron 1979; McDonnell et al. 1983). Pulmonary artery pressure is a function of pulmonary venous pressure, pulmonary vascular resistance, and cardiac output [(Pulmonary artery pressure = Pulmonary venous pressure + (Pulmonary vascular resistance × Cardiac output)]. Therefore, pulmonary hypertension is not uncommon in patients with liver disease because of their poor left ventricular compliance, increased pulmonary vascular resistance, and increased pulmonary blood flow from portosystemic shunting. The pathophysiology and management of pulmonary hypertension are well-described in Chap. 10, “Hepatopulmonary Syndrome and Portopulmonary Hypertension”.
Portal hypertension is caused by an increased intrahepatic vascular resistance and increased splanchnic blood flow. Endothelin-1, a powerful vasoconstrictor produced by the sinusoidal endothelial cells, is known to increase intrahepatic vascular resistance and activates stellate cells, and its level increases as cirrhosis progresses (Kojima et al. 2002; Gandhi et al. 1996). Normally, vasodilatory compounds, such as nitric oxide, counterbalance the increased intrahepatic vascular resistance induced by endothelin. In liver cirrhosis, however, nitric oxide production is inhibited by caveolin-1, a hepatic membrane protein that binds with endothelial nitric oxide synthase.
Pulmonary System
Hypoxemia of varying severity is present in 45–69 % of patients with significant liver disease (Krowka and Cortese 1985). The common causes are pleural effusions, impaired diffusion capacity, arteriovenous shunting, atelectasis caused by ascites or diaphragmatic dysfunction, aspiration secondary to encephalopathy, and deconditioning (Hourani et al. 1991). Ventilation–perfusion mismatch, pulmonary vasodilation, and infection also contribute to hypoxemia. Mild forms of hypoxemia are most common, although moderate-to-severe hypoxemia may be found in patients with advanced liver disease complicated by adult respiratory distress syndrome (ARDS), infection, and multiple organ failure.
Hepatopulmonary syndrome , first described by Fluckiger in 1884 (Fluckiger 1884), may cause severe hypoxemia in a subset of patients with liver disease. The syndrome consists of a triad of liver dysfunction, severe hypoxemia (PaO2 < 70 mmHg in room air), and pulmonary vasodilation, and is characterized by dyspnea, cyanosis, clubbing of the digits, exercise desaturation, and orthodeoxia (hypoxemia in upright position). Other concomitant clinical signs are a markedly increased alveolar–arterial oxygen gradient, portal hypertension, and vascular abnormality such as spider angioma and pulmonary vasodilation. The pulmonary vascular dilation (from 8–15 μ to 15–100 μ) at the precapillary level is believed to be the main pathology of the hepatopulmonary syndrome, which is caused by decreasing erythrocyte transit time and impairing diffusion of oxygen to the erythrocytes at the center of the bloodstream (Genovesi et al. 1976). In contrast with other pulmonary diseases, oxygenation improves dramatically with a high inspired oxygen concentration (FiO2), because a high alveolar concentration of oxygen overcomes the diffusion barrier and oxygenates the erythrocytes in the center of the bloodstream. The pathophysiology and management of hepatopulmonary syndrome are described in Chap. 10, “Hepatopulmonary Syndrome and Portopulmonary Hypertension”.
Non-cardiogenic pulmonary edema occurs in 37–79 % of patients with advanced liver disease, particularly in those with fulminant hepatic failure, and appears to be associated with sepsis and a neurogenic mechanism. The presence of this complication is ominous: Matuschak and Shaw reported that all 29 patients who developed non-cardiogenic pulmonary edema died before liver transplantation (Matuschak and Shaw 1987). In contrast, a rapid reversal of ARDS after liver transplantation has been reported (Doyle et al. 1993). Pulmonary edema caused by fluid overload responds to diuretics and has a relatively benign course.
Pleural effusions are found on chest X-rays in about 10 % of patients. These are caused by the unidirectional passage of ascites via diaphragmatic defects into the pleural space. Diagnostic thoracentesis is necessary to confirm the transudative nature and to exclude infection, malignancy, or embolic disease. Optimal control of ascites may prevent symptomatic pleural effusions, and transjugular intrahepatic portosystemic shunt (TIPS) is effective in treating refractory hydrothorax in 84 % of patients (Siegerstetter et al. 2001).
Renal System
Approximately 10 % of hospitalized cirrhotic patients with ascites develop the hepatorenal syndrome, which is a form of acute pre-renal kidney injury caused by circulatory dysfunction secondary to an imbalance between circulating vasodilatory and vasoconstrictive substances. The primary contributing factor for the hepatorenal syndrome is nitric oxide-induced vasodilation of the splanchnic vascular bed causing systemic arterial underfilling and relative hypovolemia (Arroyo et al. 1996). This relative hypovolemia activates baroreceptor-mediated sympathetic and the renin–angiotensin system to constrict all vascular beds including the renal vasculature (Guevara et al. 1998). The initial prostaglandin-mediated compensatory renal vasodilation is followed by renal arterial vasoconstriction and renal hypoperfusion. A striking feature of the hepatorenal syndrome is the lack of any histologic change and its reversibility: the affected kidneys resume their function after successful liver transplantation. The renal failure may be rapid (Type 1) or insidious (Type 2) and results in sodium and water retention and dilutional hyponatremia. Since the hepatorenal syndrome is a functional renal failure, the urine is similar to that found in pre-renal azotemia: oliguria, low urinary sodium, and an increased urine osmolality and urine to plasma osmolality ratio.
The major criteria for the diagnosis of the hepatorenal syndrome are as follows: (1) advanced hepatic disease and portal hypertension; (2) low glomerular filtration rate (serum creatinine >1.5 mg/dL or creatinine clearance <40 mL/min); (3) absence of nephrotoxic drug use, shock, systemic infection, or recent fluid losses; (4) lack of sustained improvement after diuretic withdrawal and volume resuscitation with 1.5 L of normal saline; (5) proteinuria (<500 mg/dL); and (6) no ultrasound evidence of urinary obstruction or parenchymal disease. Minor criteria include oliguria (<500 mL/day), urinary sodium <10 mEq/L, urinary osmolality greater than plasma osmolality, urinary red blood cells (RBCs) <50/hpf, and serum sodium <130 mEq/L. It is noteworthy that conventional renal function tests, such as BUN and creatinine levels, overestimate renal function in patients with liver failure because malnutrition and muscle wasting contribute to a low creatinine level and liver dysfunction impairs urea synthesis.
The hepatorenal syndrome is treated with the administration of vasopressin-1 agonists (i.e., terlipressin), TIPS, and, most reliably, liver transplantation. One uncontrolled trial using terlipressin with albumin for a median duration of 26 days (range 8–68 days) showed improvement in serum sodium as well as a decrease in the creatinine level below 2 mg/dL (Mulkay et al. 2001). Hemodialysis is a temporary measure and its efficacy is not reliable. The only primary preventive measure showing some promise is the administration of albumin along with antibiotics as soon as the presence of spontaneous bacterial peritonitis is diagnosed; this possibly works by preventing hypovolemia and subsequent activation of vasoconstrictor systems.
Coagulation System
All phases of hemostasis are impaired in patients with liver disease, including clot formation, fibrinolysis, and their inhibitory processes. Thrombocytopenia is found in 30–64 % of cirrhotic patients, and platelet count is commonly below 75,000/mm3. Thrombocytopenia is primarily caused by splenomegaly associated with portal hypertension, which pools up to 90 % of platelets in the spleen. However, the degree of thrombocytopenia does not closely correlate with the size of the spleen. Impaired hepatic synthesis of thrombopoietin also leads to thrombocytopenia. Thrombopoietin is involved in the maturation and formation of platelets, and its return to a normal level coincides with a gradual increase in platelet count by the fifth day after liver transplantation (Kawasaki et al. 1999). Other contributing factors are increased destruction of platelets by immune mechanisms, excessive activation of coagulation, and direct bone marrow suppression by toxins such as ethanol and folate deficiency. Additionally, platelet dysfunction is common, as demonstrated by impaired platelet aggregation to adenosine diphosphate (ADP), collagen, and thrombin (Rubin et al. 1979).
The liver produces all coagulation factors except for von Willebrand factor. Therefore, plasma levels of clotting factors are directly related to the severity of liver disease, and prothrombin time (PT) is considered to be one of the most sensitive hepatic synthetic function tests. The plasma fibrinogen level, being an acute-phase reactant, typically is normal or increased in chronic liver disease. A reduction in the fibrinogen level may indicate either a greatly reduced hepatic reserve or significant extravascular loss to ascites. Markedly prolonged thrombin time indicates the presence of dysfibrinogenemia in some patients. Dysfibrinogenemia is characterized by an excessive number of sialic acid residues in the fibrinogen molecule and abnormal polymerization of fibrin monomers. Its clinical significance is unclear.
Patients with liver disease have a tendency to develop fibrinolysis due to decreased hepatic clearance of plasminogen activators, especially tissue plasminogen activator (tPA), and reduced production of α2-antiplasmin and thrombin activatable fibrinolysis inhibitors (Van Thiel et al. 2001). Elevated levels of d-dimers, fibrin degradation products, and plasminogen are present in ascitic fluid, indicating that absorption of ascitic fluid may contribute to the hyperfibrinolysis.
On the other hand, excessive activation of coagulation is common in liver disease because of inadequate hepatic clearance of activated coagulation factors, reduced level of coagulation inhibitors, and enlarged vascular beds. The hypercoagulable state may lead to localized or disseminated intravascular coagulation (DIC), particularly in the presence of sepsis, trauma, or major surgery. The diagnosis of excessive activation of coagulation is based on the presence of a known triggering factor and the progressively worsening of coagulation with thrombocytopenia.
Anesthesia Consultation
An anesthesia consultation is performed once a patient with ESLD is referred to the liver transplantation center. The type of liver disease is identified because patients with hepatocellular disease may have more pronounced hepatic dysfunction than those with cholestatic disease or hepatocellular cancer, and certain types of liver disease may affect other vital organ function (i.e., hemochromatosis, familiar amyloidosis, etc.). The anesthesia consultation is focused on evaluation of the functional reserve of extrahepatic organs, and various tests or specific consultations may be requested (Table 2).
Table 2
Liver transplantation evaluation at the Thomas Jefferson University Hospital
![]() |
Cardiovascular Assessment
Cardiovascular assessment is performed to determine two things: (1) whether a patient can be expected to survive the operation and immediate postoperative period; and (2) whether transplantation in patients with severe cardiopulmonary disease would be futile and an inappropriate use of a scarce donor organ (Lentine et al. 2012). A suggested strategy for cardiac assessment is shown in Fig. 3 (Raval et al. 2011). Overall cardiac performance is evaluated by transthoracic echocardiography to assess myocardial contractility, abnormality in cardiac anatomy, intracardiac or intrapulmonary shunting, and pulmonary artery pressure. Most patients over age 50 years undergo non-invasive stress testing because they may have multiple CAD risk factors (i.e., diabetes, hypertension, hyperlipidemia, and pre-existing cardiovascular disease), and limited physical activity masks underlying ischemic heart disease. An exercise stress test may not be feasible in many patients with advanced liver disease, and dobutamine stress echocardiography (DSE) is commonly used, although adenosine or dipyridamole may be used when dobutamine-induced tachycardia is not desirable. DSE, with its high sensitivity and specificity, appears to be the most reliable screening test (Plotkin et al. 1998), and dobutamine-induced tachycardia may mimic intraoperative stress on the cardiovascular system. On the contrary, DSE has been reported to have poor sensitivity (as low as 13 %) and negative predictive value (as low as 75 %) (Harinstein et al. 2008), and its results may not correlate with adverse cardiac events within 30 days after transplantation (Safadi et al. 2009).


Fig. 3
Suggested strategy for preoperative cardiac assessment of liver transplantation candidates (Reprinted from JACC, 58, Raval Z, Harinstein ME, Skaro AI et al., Cardiovascular risk assessment of the liver transplant candidate, 223–231, Copyright (2011), with permission from Elsevier)
Cardiac CT scan is a non-invasive technique measuring calcium deposits within the coronary vasculature. The total amount of calcium, adjusted to the age and gender of the patient, is reported as a calcium score. High scores suggest a greater potential for coronary artery stenosis (Shaw et al. 2003; O’Rourke et al. 2000), and a calcium score of >400 has a predictive value of cardiac complications within 1 month after transplantation (Kemmer et al. 2014). This test, however, may have limited predictive value as a single screening study for CAD. Cardiac CT angiography is an alternative to invasive coronary angiography. It does appear to have negative predicting value of 100 % for clinical coronary events in patients undergoing liver transplantation (Cassagneau et al. 2012) but may not be suitable for the diagnosis of obstructive lesions at this time.
Because of the difficulty in diagnosing CAD using non-invasive testing methods, coronary angiography is recommended for patients with a positive DSE or multiple high-risk factors to identify the degree and type of obstruction. In addition, coronary angiography should be able to detect non-obstructive lesions (coronary artery stenosis <50 %), which are unlikely to be detected by stress tests but can be responsible for acute coronary syndromes (unstable angina, myocardial infarction, or sudden cardiac death) (Rubin et al. 1994; Gulati et al. 2009). Cardiac catheterization, however, can be difficult in patients with severe liver disease due to bleeding complications and the increased risk of contrast-induced nephropathy (Sharma et al. 2009).
If significant coronary artery stenosis (>70 % stenosis) is detected, revascularization may be attempted before liver transplantation. Bare metal stents are favored over drug-eluting stents to avoid the need for long-term antiplatelet therapy (6 weeks vs. 1 year). When angioplasty is not amenable, coronary artery bypass grafting (CABG) is performed. It is clear that 1-year survival after CABG is greater in patients with Child-Pugh Class A (80 %) than with Child-Pugh Class B (45 %) and C (16 %) (Filsoufi et al. 2007). Therefore, patients with Child-Pugh Class A can undergo CABG relatively safely while waiting for liver transplantation. On the other hand, patients with Child-Pugh Class B and C may require simultaneous CABG and liver transplantation.
Patients with mild-to-moderate valvular disease undergo liver transplantation without excessive complications. Similar to that of CABG, mortality after corrective valvular surgery depends on the severity of liver disease. Therefore, Child-Pugh Class C patients with severe aortic or mitral valve stenosis may undergo percutaneous balloon valvuloplasty or simultaneous valve replacement with cardiopulmonary bypass and liver transplantation.
Myocardial disease is commonly detected by transesophageal echocardiography (TEE). Patients with chronic cardiomyopathy may have attenuated systolic contraction and diastolic relaxation, altered repolarization, and reduced cardiac response to β stimulation (Liu et al. 2002). Patients with moderate-to-severe cardiomyopathy may be excluded from candidacy. Moderate-to-severe hypertrophic obstructive cardiomyopathy (HOCM) can cause left ventricular outflow obstruction by systolic anterior motion (SAM) of the anterior leaflet of mitral valve, especially in the presence of tachycardia, hypovolemia, and β stimulation. In these patients, preload and afterload should be optimized under the guidance of TEE, and tachycardia and β stimulation should be avoided. Surgical correction of the condition should be considered before or at the time of liver transplantation.
Pulmonary Evaluation
For pulmonary evaluation, results of chest X-ray, arterial blood oxygen tension in 100 % oxygen, and spirometry are reviewed to identify the degree of pulmonary shunting, obstructive, or restrictive disease. When the hepatopulmonary syndrome is suspected, contrast TEE or TC-99 m macro aggregated albumin scintigraphy may be performed for its definitive diagnosis (Krowka et al. 2000).
Renal Function
For evaluation of renal function, results of BUN, creatinine, glomerular filtration rate, levels of serum and urine electrolytes, urine output, and renal ultrasound are reviewed. The diagnosis criteria of the hepatorenal syndrome have been described earlier. In patients with chronic renal failure, simultaneous liver and kidney transplantation is performed, the criteria for which are end-stage renal disease with dialysis, no dialysis but a glomerular filtration rate <30 mL/min and proteinuria >3 g/day with a 24-h urine protein/creatinine ratio >3, and acute kidney injury requiring dialysis at least twice per week for more than 6 weeks (Charlton et al. 2009).
Fulminant Hepatic Failure
In patients with fulminant hepatic failure, reversibility of the neurologic function should be investigated using clinical signs, EEG, brain CT scan, the cerebral metabolic rate of oxygen, and TCD. In addition, ICP monitoring is recommended when a high ICP (>25 mmHg) is suspected, although its benefit should be weighed against potential complications (Vaquero et al. 2005). Poor prognostic indicators of fulminant hepatic failure are progressive hepatic failure for 7–14 days, grade 3–4 encephalopathy, intracranial hypertension, cerebral swelling, severe coagulopathy, rapid shrinkage of the liver, metabolic acidosis, hemodynamic instability, and sepsis.
Coagulation System
For the coagulation system, PT, activated partial thromboplastin time (aPTT), and platelet count are reviewed. In general, no specific coagulation therapy is requested because of the potential long waiting period and fluid overloading. Abdominal magnetic resonance imaging (MRI) is reviewed to assess the degree of portosystemic shunting and anatomy of hepatic vasculature. Additional consultation may be requested from various specialists to identify the type and severity of the specific organ dysfunction.
Contraindications
After the evaluation, all information of the potential recipient is compiled to stratify whether the patient’s condition can be optimized or meet the criteria of contraindications. Contraindications are diseases or conditions patients could have that may not improve survival after liver transplantation. They include malignancy with poor prognosis, active bacterial and viral infection, severe cardiopulmonary dysfunction, and technical difficulties. Active alcoholism is a contraindication, although demonstrable abstinence for 6 months is considered acceptable. The presence of multiple organ dysfunction is a relative contraindication for liver transplantation as the 2-year survival is approximately 25 %. Indications and contraindications of liver transplantation, however, have evolved over the past 50 years, and further modifications are expected to occur.
Candidate Selection
Anesthesiologists participate in the Transplantation Candidate Selection Committee for discussion of the hepatic disease, its complications, and the extrahepatic organ function of each patient. Once the patient is placed on the active candidate list, the United Network for Organ Sharing (UNOS) is notified and the patient is given a MELD (Model for End-Stage Liver Disease) or PELD (Pediatric End-Stage Liver Disease) score for fair distribution of donor livers.
Surgical Aspects of Liver Transplantation
Although surgical techniques are fully described elsewhere, a brief description of their physiologic effects is warranted here.
In OLT, after removal of the diseased liver, the donor liver is placed anatomically in the right upper quadrant. For the convenience of description, the procedure is divided into three stages: stage 1 (dissection stage), stage 2 (anhepatic stage), and stage 3 (neohepatic stage). The dissection stage begins with an inverted Y-shaped bilateral subcostal skin incision and ends with the skeletonization of the diseased liver. The anhepatic stage begins with the occlusion of the hepatic artery, portal vein, and IVC for hepatectomy. However, the patient is virtually anhepatic once the hepatic artery or portal vein is occluded. Three surgical techniques are used for hepatectomy and vascular reconstruction during the anhepatic stage: OLT with simple venous cross-clamping, OLT with venovenous bypass, and the piggyback technique.
Orthotopic Liver Transplantation (OLT) with Simple Venous Cross-Clamping
In OLT with simple venous cross-clamping, the diseased liver is removed together with the retrohepatic portion of the IVC after cross-clamping of the suprahepatic and infrahepatic IVC, hepatic artery, and portal vein (Fig. 4). After surgical hemostasis of the hepatic bed, the donor liver is placed in the right upper quadrant, and sequential anastomoses of the suprahepatic IVCs, infrahepatic IVCs, portal veins, and hepatic arteries are performed. During the infrahepatic IVC anastomosis, the liver allograft is flushed with 1000 mL of cold lactated Ringer’s solution or 5 % albumin solution through a cannula in the portal vein. This flush technique allows preservation solution, metabolites, and air in the donor liver to escape through the incompletely anastomosed infrahepatic IVC. A second flush may be used by allowing 300–500 mL of blood to escape through the incompletely anastomosed portal vein by unclamping the infrahepatic IVC (back-bleeding technique). When the portal vein of the recipient is less than optimal, the superior mesenteric vein, collateral vein, or venous graft may be used for portal blood supply. The hepatic artery is reconstructed by end-to-end hepatic arterial anastomosis. However, an arterial graft is placed between the graft hepatic artery and the infrarenal aorta of the recipient with a side clamp on the aorta when the size or anatomy of the recipient hepatic artery is less than optimal.


Fig. 4
Schematic diagram of three surgical techniques during the anhepatic stage. Line thickness indicates relative blood flow to each vasculature. The simple cross-clamping technique reduces venous return and cardiac output and leads to congestion of the viscera, kidneys, and lower extremities. The venovenous bypass technique maintains venous return without visceral congestion. Hemodynamic changes in the piggyback technique are between the two other techniques. HA hepatic artery, HV hepatic vein, IVC inferior vena cava, PV portal vein, RV renal vein)
The liver is reperfused by the sequential unclamping of the infrahepatic IVC, portal vein, suprahepatic IVC, and hepatic artery. After hemostasis, choledochocholedochostomy is performed frequently with a T-tube. Choledochojejunostomy using a Roux-en-Y loop is performed when the bile ducts are diseased or mismatched in size. The abdomen is closed once the absence of foreign bodies in the peritoneal cavity is confirmed. In patients with a large graft or swollen intestine, the abdomen may require secondary closure.
OLT with Venovenous Bypass
OLT with venovenous bypass was developed in 1983 to minimize reduction of venous return associated with the cross-clamping of the IVC and portal vein by diverting blood from the IVC and portal vein to the axillary vein using a centripetal magnetic pump (Shaw et al. 1984). Once the hepatic hilum is dissected, cannulas are inserted into the left superficial femoral vein (7 mm) and portal vein (9 mm) for outflow from the patient and into the left axillary vein (7 mm) for venous inflow. The cannula site and size may vary depending on the preference of the surgical team or anatomic variations. The cannulas and heparin-bonded tubings are flushed with heparin solution (2000 U/L) to avoid thrombosis during preparation. Systemic heparinization is not used because of the presence of pre-existing coagulopathy and the use of heparin-bonded tubings. The bypass run begins by unclamping all cannulas while the pump speed is gradually increased to achieve the maximal flow rate. Hepatectomy and anastomoses of the suprahepatic and infrahepatic IVC are performed once full bypass is achieved. The removal of the portal cannula for portal venous anastomosis leads to a partial bypass, which reduces venous return. Bypass is terminated after the engrafted liver is reperfused, and cannulas are removed.
The advantages of venovenous bypass are (1) well-preserved cardiac output by uninterrupted venous return from the viscera and lower extremities; (2) effective decompression of the portal venous system, which decreases bleeding and intestinal congestion; (3) avoidance of renal congestion, oliguria, and hematuria; and (4) simplified anhepatic stage allowing meticulous hepatectomy and vascular anastomoses. Long-term complications are neurovascular injury, thrombosis, infection, lymphocele, and seroma at the cannulation sites.
As an alternative to the traditional venovenous bypass technique, percutaneous cannulation was introduced. In this technique, inflow to the patient is achieved by percutaneous cannulation of the right internal jugular vein (16–20 French) performed by the anesthesia team using a Seldinger technique, and outflow from the patient by percutaneous cannulation of the left femoral vein (16–20 French) and a portal cannula by the surgical team. This technique is generally safe, but the inadvertent extravascular placement of an inflow cannula may cause a massive hemothorax (Sakai et al. 2007).
Piggyback Technique
The piggyback technique was originally designed for patients with significant cardiovascular disease, portacaval shunt, superior vena caval syndrome, or small donor livers (Tzakis et al. 1989). In this technique, the diseased liver is removed without the retrohepatic portion of the IVC by peeling the diseased liver off the IVC after transaction of the hepatic veins, hepatic artery, and portal vein. Therefore, systemic venous return can be relatively well preserved via the intact IVC during the anhepatic stage. Vascular anastomoses are made between the reconstructed ostia of the recipient by combining hepatic veins and the suprahepatic IVC of the graft for the drainage of the hepatic venous blood. The portal vein of the recipient and the graft are anastomosed for portal blood supply, and the infrahepatic IVC of the graft is ligated.
The neohepatic stage begins with reperfusion of the grafted liver by sequential unclamping of the infrahepatic IVC, portal vein, suprahepatic IVC, and hepatic artery, although the sequence of unclamping may vary depending on the surgical technique. Reperfusion is followed by hepatic arterial anastomosis (if it has not been performed already), biliary reconstruction, and closure of the abdomen.
Preparation and Anesthetics
Immediate preoperative consultation is made when a donor organ is identified. The patient is re-evaluated to identify any interval changes during the waiting period. Anesthetic and postoperative management and their risks are explained to the patient one more time. In general, pre-medication is withheld in most cases because of potential encephalopathy and hypovolemia, and narcotics (e.g., fentanyl 1–5 μg/kg) are commonly administered intravenously in the operating room.
Necessary medications and anesthesia equipment are listed in Table 3. A device that delivers fluids and blood rapidly on demand is considered standard equipment (i.e., FMS2000® fluid warming system, Belmont Instrument Corp., Billerica, MA, USA) (Elia and Kang 2002). An autotransfusion system is helpful in minimizing the need for bank blood (Dzik and Jenkins 1985; Kang et al. 1991). A system that monitors coagulation , either a conventional coagulation profile, Thromboelastography R with circle (TEG; Haemonetics, Braintree, MA, USA), or Rotational Thromboelastometry R with circle (ROTEM; TEM International, Basel, Switzerland), is essential in monitoring and management of coagulation (Kang 1986, 1997). TEG and ROTEM provide similar physical properties of blood coagulation, although TEG monitors shear elasticity and ROTEM monitors viscoelasticity. In general, 20 units each of cross-matched packed RBCs (PRBCs) and fresh frozen plasma (FFP) are available at all times, and 10 units of each are prepared in the operating room. Platelets (10–20 units) should be available on demand.
Table 3
Anesthesia equipment and medications for liver transplantation
Equipment |
Anesthesia machine with volume ventilator |
Mass spectrometer or capnograph |
Multiple-channel vital-sign monitor |
Pulse oximeter |
Cardia output computer (oximetry or right ventricular ejection fraction) |
Transesophageal echocardiograph |
Drug infusion pumps |
Thromboelastograph or thromboelastometer |
Rapid-infusion device |
Autotransfusion system |
Warming blanket |
Defibrillator |
Medications |
Induction agents |
Propofol |
Etomidate |
Intravenous agents |
Midazolam |
Fentanyl |
Inhalation agents |
Isoflurane |
Muscle relaxants |
Succinylcholine |
Rocuronium |
Vecuronium |
Other drugs |
Atropine (0.4 mg) |
Calcium chloride (100 mg/mL, 200 mL) |
NaHCO3 (40 mmol/50 mL, 400 mmol) |
Tromethamine (THAM) (500 mL, 1500 mL) |
Ephedrine (5 mg/mL, 20 mL) |
Epinephrine (4 μg/mL, 20 mL) |
Epinephrine (40 μg/mL, 20 mL) |
ε-Aminocaproic acid (EACA) (250 mg/mL, 10 mL) |
Protamine (10 mg/mL, 10 mL) |
Insulin: available in the refrigerator |
Potent vasoactive drips: as needed |
Two large-bore intravenous (IV) catheters (up to 8.5 or 9 French) are secured, typically in the right antecubital and right or left internal jugular vein. When the antecubital vein is unavailable, two catheters may be placed in the same internal jugular vein. Catheter patency is confirmed by noting the line infusion pressure of <300 mmHg during fluid infusion at 500 mL/min. Sterile technique should be followed during catheterization, and antiseptic ointment or antiseptic patch is applied at the skin puncture site. A nasogastric tube is placed with copious lubrication and topical vasoconstrictor to avoid nasal or esophageal variceal bleeding.
Proper monitoring is prerequisite to a successful outcome because patients undergoing liver transplantation develop clinically significant hemodynamic, hematologic, metabolic, and other homeostatic abnormalities. Non-invasive monitoring is similar to that for patients undergoing any major surgery. For invasive monitoring, two intra-arterial catheters (20 gauge in the left radial artery and 16–18 gauge in the right femoral artery) are used at the Thomas Jefferson University Hospital. Femoral arterial pressure monitoring is preferred because it reflects central arterial blood pressure more accurately in the presence of low systemic vascular resistance, particularly after reperfusion (Lee et al. 2015). Radial arterial pressure monitoring is useful for blood sampling and backup pressure monitoring when the aorta is partially or completely clamped during aorta-to-hepatic artery anastomosis. A pulmonary artery catheter (PA catheter) is inserted via the right internal jugular vein to monitor cardiac output, intracardiac pressures, and core temperature. Carotid artery puncture should be assiduously avoided because of the presence of coagulopathy. An oximetric-type PA catheter provides additional information on mixed venous hemoglobin oxygen saturation (SvO2). The right ventricular ejection fraction-type PA catheter monitors the right ventricular ejection fraction and right ventricular end-diastolic volume. It has been shown that central venous pressure (CVP) and pulmonary capillary wedge pressure (PCWP) are not as sensitive as right ventricular end-diastolic volume in estimating preload, particularly during the anhepatic stage (DeWolf et al. 1993b). Recently, non-invasive, continuous cardiac output monitoring was introduced; however, the technique is not reliable in monitoring cardiac output in hyperdynamic patients. In some centers, a CVP catheter is used instead of a PA catheter. This, of course, is justified if hemodynamic derangement is kept minimal during the entire surgical procedure. However, most centers use a PA catheter for three reasons: (1) hemodynamic instability can be unpredictable during liver transplantation; (2) determination of cardiac output and preload is more clinically significant than CVP monitoring; and (3) it is an important educational tool for trainees. TEE is used in all patients at the Thomas Jefferson University Hospital to monitor myocardial contractility, ventricular end-diastolic volume, wall motion abnormality, air or thromboembolism, intrapulmonary shunting, and patency of the reconstructed major veins. A TEE probe may cause esophageal variceal bleeding (Burger-Klepp et al. 2012) and it should therefore be placed gently.
Various laboratory tests are performed, including arterial blood gas tension and acid–base state, and serum level of electrolytes, ionized calcium, glucose, lactate, and ionized magnesium if available. Typical test times are before and after induction of anesthesia, every hour during the dissection stage, 5 min after the onset of the anhepatic stage, every 30 min during the anhepatic stage, 15 min before reperfusion, 5 and 30 min after reperfusion, and every hour thereafter. Coagulation is monitored by conventional coagulation profile (PT, aPTT, fibrinogen level, and platelet count) and TEG or ROTEM at the following times: before induction of anesthesia, every hour during the dissection stage, 15 and 60 min after onset of the anhepatic stage, 15 min before reperfusion, 5 and 30 min after reperfusion, and every hour thereafter.
Monitoring of TEG or ROTEM and the platelet count is preferable as a conventional coagulation profile has several drawbacks when used during liver transplantation (Kang 1995). PT is a very sensitive hepatic function test and is prolonged in most patients undergoing liver transplantation. Administration of FFP to correct the PT may not be possible or desirable in the course of surgery. aPTT follows a similar time course to PT, and its correction may not be practical. It is a sensitive test for the heparin effect, and its prolongation indicates the presence of heparin released from the bypass circuit or grafted liver. The fibrinogen level is frequently maintained within the acceptable range, although severe hypofibrinogenemia may indicate either active fibrinolysis or excessive activation of coagulation. The level of fibrin(ogen) degradation products is usually elevated in most patients due to excessive activation of coagulation and reabsorption of defibrinated blood from the abdominal cavity and does not have any immediate clinical significance. Further, coagulation profile results may not be available in a timely manner.
TEG/ROTEM has several advantages over a conventional coagulation profile and has been accepted as a standard coagulation monitoring tool by the ASA (American Society of Anesthesiologists 2015). It rapidly and reliably measures blood coagulability (quality) instead of the quantity of each coagulation component. An accurate differential diagnosis can be made for replacement therapy and pharmacologic therapy by comparing TEG/ROTEM of untreated blood with that of blood treated with various blood components (FFP, platelets, cryoprecipitate) or pharmacologic agents (protamine sulfate, heparinase, ε-aminocaproic acid [EACA], aprotinin) (Fig. 5) (Kang et al. 1987).


Fig. 5
Effects of pharmacologic agents on pathologic coagulation immediately after reperfusion (From Kang YG (1986) Monitoring and treatment of coagulation. In: Winter PM, Kang YG (ed) Hepatic Transplantation, anesthetic and perioperative management. Prager, New York, with the permission of the publisher)
Lastly, circumferential identification tags around the wrists or ankles are removed to avoid the compartment syndrome. Both arms are placed on padded arm boards in an abducted position, and excessive abduction should be avoided to prevent a plexus stretch injury. The extremities are protected with foam padding to avoid pressure injuries.
A rapid-sequence induction is preferred because of uncertain gastric emptying. Anesthesia is commonly induced with propofol (2–3 mg/kg) or etomidate (0.3 mg/kg), and fentanyl (2–5 μg/kg) is frequently added. Succinylcholine (1–2 mg/kg) or rocuronium bromide (1.2 mg/kg) is used to facilitate intratracheal intubation. Anesthesia is maintained using volatile inhalation agents and narcotics. Isoflurane is the preferred inhalation agent because its effect includes less myocardial depression and biotransformation. Nitrous oxide is avoided because it distends the bowel and increases the size of any entrained air. Midazolam (1–4 mg) may be added for amnesia. For muscle relaxation, rocuronium bromide, vecuronium bromide, or cisatracurium besilate are commonly used.
Antibiotics and immunosuppressants administered during surgery may vary from center to center. At the Thomas Jefferson University Hospital, Unasyn® (ampicillin/sulbactam 3 g) is given before incision and every 4 h thereafter. For patients allergic to cephalosporin or penicillin, vancomycin is administered within 2 h before skin incision (1 g for patients <80 kg and 1.5 g for those >80 kg). For immunosuppression, methylprednisolone (500 mg IV) and basiliximab (20 mg IV) are given during the anhepatic stage and tacrolimus is given in the postoperative period.
Physiologic Homeostasis During Liver Transplantation
Liver transplantation imposes a great deal of physiologic stress on patients, and maintenance of physiologic homeostasis is essential to a successful outcome.
Cardiovascular Homeostasis
The goal of hemodynamic management is to optimize tissue perfusion by maintaining the hyperdynamic state characteristic of ESLD. In general, there are two schools of thought about maintaining hemodynamic stability. The first endorses maintaining the hyperdynamic state to optimize cardiac output and tissue perfusion. Patients with ESLD have generalized vasodilation and are known to have oxygen debt at the tissue level. Therefore, maintaining the hyperdynamic state, instead of ‘normal blood pressure,’ ensures ample oxygen delivery to tissues and avoids tissue acidosis. This, in turn, optimizes tissue metabolism and hepatic blood flow. The second school of thought endorses maintaining arterial blood pressure within the normal range. This may include the use of various vasopressors (phenylephrine, norepinephrine, vasopressin, etc.) with or without hypovolemia. In extreme cases, blood is removed from the patient to induce hypovolemia, and blood pressure is supported by vasopressors (Massicotte et al. 2010); it has been claimed that blood loss is minimal without increasing perioperative complications, although fluid restriction may lead to tissue ischemia, renal failure, and air embolism (Melendez et al. 1998; Schroeder et al. 2004). Further, α-vasopressors (norepinephrine and phenylephrine) decrease hepatic blood flow by reducing portal venous flow dramatically in the presence of a limited hepatic arterial buffer response (Mehrabi et al. 2005).
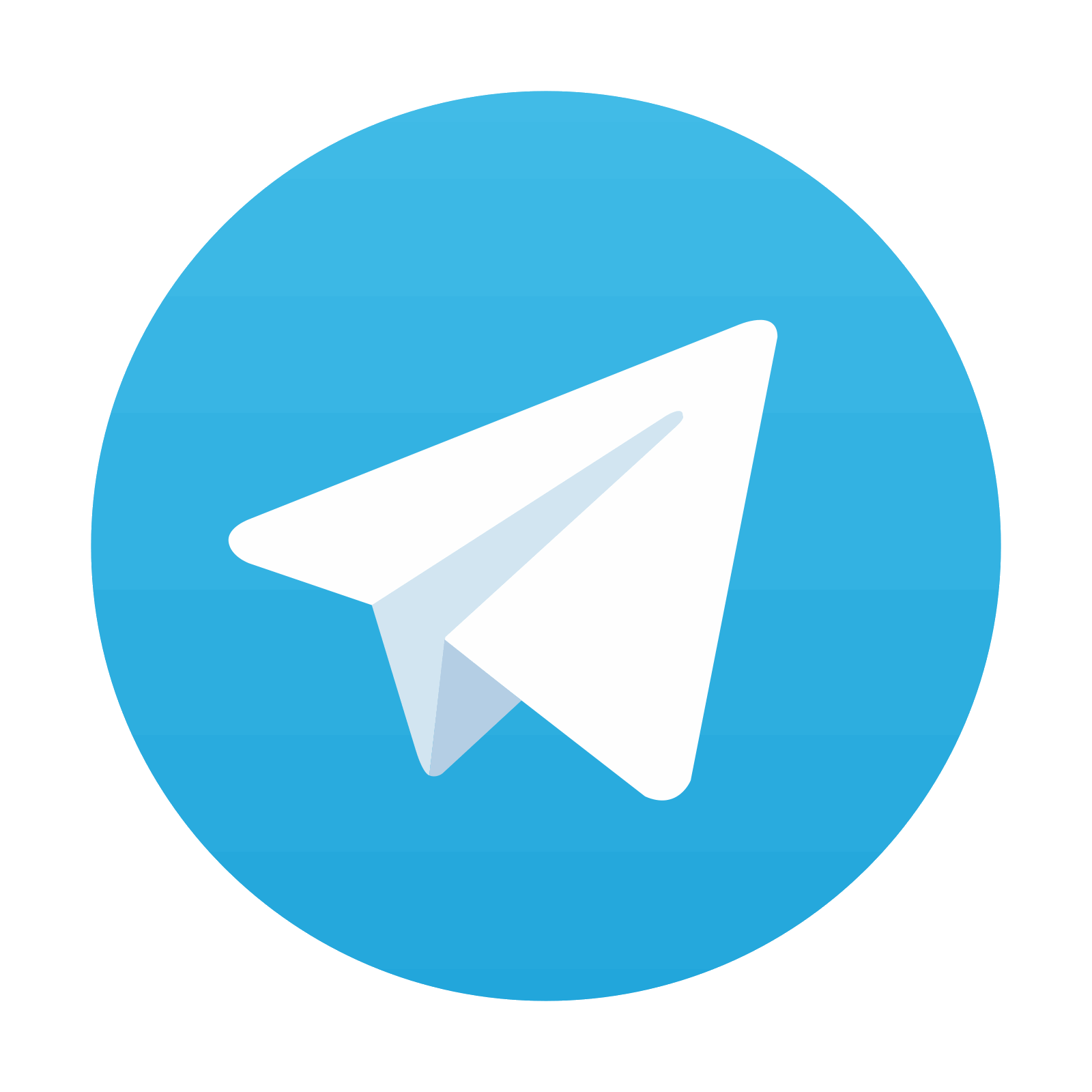
Stay updated, free articles. Join our Telegram channel
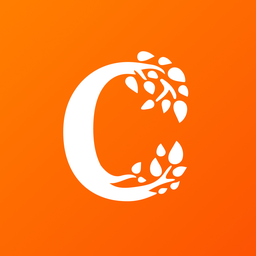
Full access? Get Clinical Tree
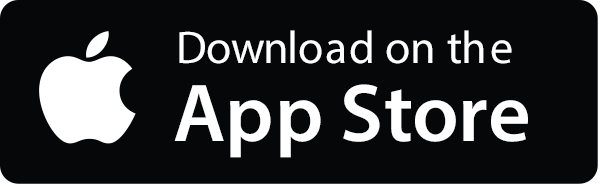
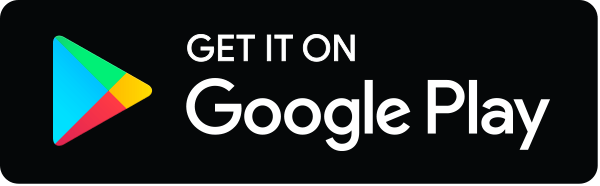