80% to 85% of filtered HCO3– is reabsorbed in the proximal tubules; 15% to 20% is reabsorbed in the thick ascending loop of Henle; ˜5% is reabsorbed in the distal nephron. Although the amount of distal HCO3– reabsorption is small, this can increase in proximal renal tubular acidosis (pRTA).
FIGURE 2.1 Bicarbonate reabsorption in the proximal tubule. 80% to 85% of HCO3– is reabsorbed in the proximal tubules. 1. The Na+-H+ antiporter NHE3 and, to a lesser extent, H+-ATPase secrete H+ into the lumen. 2. Secreted H+ binds filtered HCO3– to form carbonic acid (H2CO3). 3. CA IV catalyzes the dissociation of H2CO3 into H2O + CO2. 4. CO2 diffuses into the cell, combines with H2O (which enters the cell via AQP1) to reform H2CO3 (via the catalytic activity of CA II), then again redissociates to H+ + HCO3–. 5. H+ is resecreted into the lumen, whereas HCO3– is reabsorbed via the Na+-HCO3– cotransporter NBC1. Abbreviations: AQP1, aquaporin 1; CA II, carbonic anhydrase II; CA IV, carbonic anhydrase IV; NBC1, sodium bicarbonate cotransporter-1; NHE3, sodium hydrogen exchanger-3.
The Na+-H+ antiporter sodium hydrogen exchanger-3 (NHE3) and, to a lesser extent, H+-ATPase secrete H+ into the lumen where it binds filtered HCO3– to form carbonic acid (H2CO3). Carbonic anhydrase IV (CA IV) catalyzes the dissociation of H2CO3 into H2O + CO2. CO2 diffuses into the cell and combines with H2O to reform H2CO3, which redissociates into H+ + HCO3–. H+ is resecreted into the lumen, whereas HCO3– is reabsorbed via the sodium bicarbonate cotransporter-1 (NBC1).
In acute metabolic acidosis, cytoplasmic NBC1 is recruited into basolateral membranes to enhance HCO3– reabsorption.
In metabolic alkalosis, NBC1 is redistributed into the cytoplasm to reduce HCO3– reabsorption.
In respiratory acidosis, NBC1 protein synthesis is upregulated.
H+ secretion occurs predominantly in the late distal tubule, connecting segment, and cortical and medullary collecting tubules at approximately 1 mEq/kg/d, reflecting daily (dietary) acid load.
FIGURE 2.2 H+, HCO3–, and K+ handling in the collecting duct. Sodium delivery to the collecting duct enters the principal cells via the apical ENaC. The reabsorbed Na+ is subsequently reabsorbed on the basolateral side in exchange for intracellular K+ uptake via 3Na+-2K+-ATPase. The increase in intracellular K+ gives rise to a favorable chemical gradient for K+ secretion. The apical uptake of Na+ also creates an electronegative lumen that favors the secretion of positively charged K+ via ROMK (in principal cells) and H+ via H+-ATPase and, to a lesser extent, H+-K+-ATPase by type A-intercalated cell (α-intercalated cells). Hypokalemia increases H+-K+-ATPase activity. Abbreviations: α-IC, α-intercalated cells; β-IC, β-intercalated cells; CA II, carbonic anhydrase II; ENaC, epithelial sodium channel; ROMK, renal outer medullary potassium channel.
Sodium enters the principal cells via the apical amiloride-sensitive epithelial sodium channel (ENaC).
The reabsorbed Na+ is subsequently reabsorbed on the basolateral side in exchange for intracellular K+ uptake via 3Na+-2K+-ATPase. The increase in intracellular K+ gives rise to a favorable chemical gradient for K+ secretion.
The apical uptake of Na+ also creates a relative electronegative lumen that favors the secretion of positively charged K+ via the renal outer medullary potassium channel (ROMK in principal cells) and H+ via H+-ATPase and, to a lesser extent, H+-K+-ATPase in type A-intercalated cells (α-intercalated cells or α-IC).
Aldosterone stimulates both H+ and K+ secretion by increasing the expression of ENaC and Na+-K+-ATPase and increasing the activity of ROMK.
The efficiency of H+ secretion also depends in part on the presence of ammonia (NH3), which serves to buffer the secreted H+ and facilitate urinary excretion.
H+ + NH3 → NH4+ (excreted into urine)
Optimal H+ and K+ secretions require adequate Na+ delivery to the distal nephron, intact ENaC activity, presence of aldosterone, and good urine flow.
Potassium-depleted state enhances the activity of H+-K+-ATPase, which serves to increase K+ reabsorption in exchange for H+ secretion. (This partly explains how metabolic alkalosis may be present in association with severe hypokalemia.)
Cortical collecting ducts (CCDs) have type B-intercalated cells (β-IC), which are thought to be mirror image of α-IC in terms of H+/HCO3– secretion.
β-IC secrete HCO3– in exchange for Cl– into the lumen via the apical chloride-bicarbonate exchanger (known as pendrin) while reabsorbing H+ on the basolateral cell surface. In contrast, α-IC secrete H+ into the lumen while reabsorbing HCO3– on the basolateral side.
Depending on the acid-base status, distal tubules can switch intercalated cell types to secrete either H+ or HCO3–. Chronic metabolic acidosis increases the proportion of α-IC, whereas metabolic alkalosis increases the proportion of β-IC. Extracellular matrix proteins (e.g., hensin, galectin-3, and others) along the intercalated cell basolateral surface have been suggested to play a role in this cell-type expression switch.
The substrate for ammoniagenesis is glutamine.
Glutamine is taken up by proximal tubular cells via the sodium-dependent amino acid transporters (SNAT3), where NH4+ is formed in a multistep process (Fig. 2.3):
Rate-limiting enzymes for ammoniagenesis include phosphate-dependent glutaminase (GA) and phosphoenolpyruvate carboxykinase (PEPCK).
NH4+ is either transported as NH4+ into the lumen via NHE3 or freely diffuses into lumen as NH3. AII upregulates NHE3, thereby increasing NH4+ secretion.
Luminal NH3 and NH4+ are reabsorbed at thick ascending limb of loop of Henle (TAL) into the interstitium where they are subsequently secreted back out into the lumen at the CCD with H+ (via nonionic diffusion). NH4+ may
be reabsorbed at TAL via the Na+-K+-2Cl– cotransporter (NKCC2). Secretion of NH3 at the connecting segments and collecting tubules is facilitated by the nonerythroid glycoproteins RhBg and RhCg (Fig. 2.4).
Ammoniagenesis and ammonia transport along the nephron are stimulated by metabolic acidosis and hypokalemia.
Metabolic acidosis and hypokalemia:
Increase ammoniagenesis by increasing the expression of involved enzymes, including GA, glutamate dehydrogenase, and PEPCK
Metabolic acidosis also:
Increases mobilization of glutamine from skeletal muscle and intestinal cells
Increases the expression of NKCC2
In the evaluation of acid-base disorders, the use of either arterial or venous blood gas (ABG and VBG, respectively) generally provides similar answers.
Nonetheless, on average, the differences in ABG versus VBG for pH, pCO2, and HCO3– (referred to as total CO2 [tCO2] in venous blood) are as follows:
pH (ABG) ≈ pH (VBG) + 0.03
FIGURE 2.4 Ammoniagenesis within the nephron. 1. NH4+ generated from glutamine in the proximal tubule is secreted into the lumen via NHE3. 2. Luminal NH4+ may be reabsorbed at the loop of Henle via NKCC2. 3. Secretion of NH3 at the connecting segments and collecting duct is facilitated by nonerythroid glycoproteins RhBg and RhCg. Abbreviations: CD, collecting duct; CN, connecting segment; NHE3, sodium hydrogen exchanger-3; NKCC2, Na+-K+-2Cl– cotransporter; PT, proximal tubule; Rhg, nonerythroid glycoproteins B and C (RhBg, RhCg); SNAT-3, sodium-dependent amino acid transporter.
pCO2 (ABG) ≈ pCO2 (VBG) – 4
HCO3– (ABG) ≈ tCO2 (VBG) – 1
The measured venous tCO2 from a routine chemistry panel is typically higher than the calculated [HCO3–] from an ABG. Although other factors may be contributory, the discrepancy between the two values has been largely explained by the fact that venous tCO2 measures HCO3–, H2CO3, and dissolved CO2 gas and pCO2 is greater in venous blood.
Notable conditions with high serum anion gap (SAG) metabolic acidosis to know well for the Boards:
Propylene glycol is used as a solvent in a number of medications (e.g., topical sulfadiazine silver cream, intravenous nitroglycerin, multivitamins, phenytoin, lorazepam, diazepam, etomidate, enoximone).
Propylene glycol is metabolized to L-lactate, hence high SAG metabolic acidosis.
Suspect propylene glycol induced lactic acidosis when there is a case of sepsis-like presentation with high SAG metabolic acidosis and high lactic acid levels, but absence of bacterial sepsis (e.g., alcoholic patient receiving high infusion of lorazepam; patient with severe burns receiving high amount of sulfadiazine cream).
Other notable signs/symptoms associated with high propylene glycol accumulation: high serum osmolality, hemolysis, arrhythmias, hypotension, multiorgan failure, seizures, coma
Management: supportive therapy, offending drug withdrawal
Ingestion of ethylene glycol (present in antifreeze) may result in kidney failure due to metabolism to oxalate and precipitation of calcium oxalate. See Figure 3.11 for calcium oxalate crystals.
Chronic acetaminophen use in malnourished chronically ill patients may be associated with high SAG metabolic acidosis due to excess production of oxoproline (OXO). This is due to the lack of negative feedback from glutathione as glutathione is also used in the metabolism of acetaminophen.
Notable findings other than high SAG metabolic acidosis:
Acetaminophen level may be low or negative (as this is associated with long-term use of acetaminophen, not acute overdose). In contrast, in acute acetaminophen poisoning, acetaminophen level will be high, and lactate level may be high.
Absence or low serum levels of lactate or ketones that could normally explain increased SAG
Management: fluid support; consider acetylcysteine therapy to replete glutathione.
Lactic acid stereoisomer: L-lactate
Underlying cause: tissue hypoperfusion, hypoxia (including severe anemia), carbon monoxide poisoning
Conditions associated with type A lactic acidosis: sepsis, severe prolonged hypotension, cardiogenic shock, vigorous exercise, seizures
FIGURE 2.5 Lactic acidosis. Glucose is taken up by the cell where it undergoes glycolysis to form pyruvate. Pyruvate either forms lactate via lactate dehydrogenase or enters the mitochondria where it is converted to acetyl-CoA via pyruvate dehydrogenase. Thiamine is a cofactor for pyruvate dehydrogenase. In the mitochondria, acetyl-CoA enters the tricarboxylic acid cycle, where 2 ATPs are produced. NADH and FADH2 formed from TCA are shuttled to the electron transport chain for oxidative phosphorylation, a process whereby 32 more ATPs are produced in the presence of oxygen. Type A lactic acidosis occurs under hypoxic conditions where oxidative phosphorylation is compromised by the lack of oxygen. This leads to anaerobic metabolism, accumulation of pyruvate, and subsequent conversion to lactate. Type B lactic acidosis occurs via defects/problems at any of the 6 components unrelated to the lack of oxygen.
1. Excessive glycolysis (seen in caffeine intoxication with overactive sympathetic nervous system stimulation, high-dose β2-agonist used in the treatment of asthma, pheochromocytoma, metabolically active malignant cells)
2. Lactate dehydrogenase inhibition in the conversion of lactate to pyruvate (isoniazid)
3. Inadequate thiamine (metabolically active malignant cells or severe alcoholism in combination with malnutrition)
4. Drugs/toxins leading to mitochondrial injury and/or oxidative phosphorylation (methanol, propofol, linezolid, salicylate, cyanide, mangostin)
5. Inhibition of pyruvate carboxylase: Metformin is thought to cause lactic acidosis by inhibiting oxidative phosphorylation and pyruvate carboxylase, with the latter blocking the first step of gluconeogenesis.
6. Liver failure can limit gluconeogenesis and cause accumulation of lactic acid (nucleoside reverse transcriptase inhibitors didanosine, stavudine, acute fulminant liver failure).
Abbreviations: ATP, adenosine triphosphate; ECT, electron chain transport; TCA, tricarboxylic acid cycle.
Lactic acid stereoisomer: L-lactate or D-lactate
Underlying cause: NOT associated with tissue hypoperfusion or hypoxia
Enhanced glycogenolysis, lipolysis, glycolysis
Malignancies: leukemias, lymphoproliferative malignancies, tumors involving liver metastatic disease; may be ameliorated with thiamine
Toxic levels of caffeine, β2-agonists or stimulation (e.g., treatment of asthma, pheochromocytoma); treat with β-blocker. Note: Lactic acidosis associated with the treatment of asthma with β2-agonist is benign (unless, of course, respiratory status declines, in which case, lactic acidosis is due to type A).
Diabetic ketoacidosis (DKA)
Inherited mitochondrial disease
Acquired mitochondrial dysfunction, interference of oxidative phosphorylation:
Highly active retroviral therapy (nucleoside reverse transcriptase inhibitors): didanosine, stavudine; may be treated with uridine
Mangosteen (Southeast Asian fruit) contains α-mangostin, a potent inhibitor of mitochondria function
Drugs: metformin (inhibits pyruvate carboxylase that blocks the first step of gluconeogenesis and mitochondrial function), propofol (mitochondrial injury), linezolid (inhibits mitochondrial function)
Others: toxic alcohols, methanol (formic acid can inhibit mitochondrial function and increased anaerobic metabolism), ethylene glycol, salicylates (causes uncoupling of oxidative phosphorylation in mitochondria), cyanide (mitochondrial toxin), isoniazid
Impaired pyruvate dehydrogenase activity: severe thiamine deficiency (active malignant tumors or alcoholism with malnutrition)
Impaired lactate clearance: acute fulminant liver failure
Gut bacterial overgrowth:
D-Lactic acid is produced by gut bacterial overgrowth in short-bowel syndrome. This is NOT the same as what humans predominantly produce, which is L-lactate.
Clinical manifestations:
Laboratory findings: Typically, a high SAG metabolic acidosis is seen at presentation. However, patients may present with a normal SAG metabolic acidosis (hyperchloremic metabolic acidosis). Renal reabsorption of D-lactic acid is much lower than that for L-lactic acid. The high renal excretion of D-lactate can thus lead to a normal SAG in those with D-lactate and good kidney function with high urine flow.
D-lactic acid level > 3 mmol/L.
Patients with D-lactate typically present with encephalopathy, slurred speech, ataxia, gait disturbances, mimicking a “drunk.”
D-Lactic acidosis can be exacerbated following high carbohydrate ingestion and metabolic acidosis.
Treatment: oral metronidazole or vancomycin, supportive
Diabetic ketoacidosis (DKA):
D-Lactate is derived from methylglyoxal, a metabolite of acetone and dihydroxyacetone phosphate, which are intermediates that can accumulate in DKA. D-Lactate may be elevated in severe DKA.
Propylene glycol intoxication (case report)
Ingestion or infusion of any alcohol will increase the serum osmolality because the alcohol osmoles will be measured with routine serum osmolality measurements. Whenever there is a suspicion for intoxication/poisoning, calculate SOG.
Serum osmolality gap (SOG):
BUN = blood urea nitrogen
When there is no ingestion/infusion of exogenous osmoles (e.g., alcohols), SOG < 12 mOsm/kg.
An SOG >> 12 mOsm/kg indicates the presence of osmoles that are not normally present in the serum, which may suggest ingestion/poisoning with various alcohols.
Increased SOG should raise concerns for the presence of other osmoles, that is, intoxications (ethanol, methanol, ethylene glycol, isopropanol, or toluene), OXO, ketones, and/or lactate.
If only ethanol ingestion is suspected, the concentration of ethanol (mmol/L) should match ΔSOG.
If the ethanol concentration does not fully account for the increase in normal SOG [i.e., ΔSOG > serum ethanol concentration (mmol/L)], then there must be other unknown osmoles in the blood, in which case evaluation for other concurrent ingestions such as methanol, isopropanol, and/or OXO/PA is warranted.
Note: The absence of an increased SOG does not necessarily rule out alcohol poisoning if patient presents after the parent compound has been metabolized to its toxic metabolites.
See Figure 2.6 and Table 2.1 for metabolism and management of different alcohol intoxications.
All forms of ketoacidosis (alcoholic, starvation, and DKA) occur when hepatic lipid metabolism is switched to a state of increased ketogenesis due to relative or absolute insulin deficiency.
Risks: individuals with a history of alcohol use, typically with recent history of binge drinking, poor oral intake, persistent vomiting, volume depletion
Clinical manifestations: sweet ketone breath; high SAG metabolic acidosis may be severe, pH <7; mixed acid-base disorders may be present, including metabolic alkalosis from vomiting and volume depletion.
FIGURE 2.6 Metabolism of methanol and ethylene glycol. Both methanol and glycol alcohols undergo metabolism to their respective toxic metabolites via alcohol dehydrogenase. Ethanol is also a substrate for alcohol dehydrogenase and may be used as a competitive antagonist to reduce the metabolism of methanol and glycol alcohols to their respective toxic metabolites. Ethanol is metabolized by alcohol dehydrogenase and aldehyde dehydrogenase to form acetate, which is subsequently metabolized to CO2 and H2O. Optimal efficacy of ethanol as a competitive inhibitor of alcohol dehydrogenase occurs at levels ranging between 100 and 200 mg/dL. Abbreviation: THF, tetrahydrofolate.
Table 2.1 Alcohol intoxication
Alcohol
Methanol
Ethylene Glycol
Isopropyl Alcohol
Toxic metabolite
Formate
Oxalate
Acetone
Clinical complications
Blindness
Kidney failure
CNS depression, nausea, vomiting, usually not fatal
Treatment
Fomepizole if ethylene glycol level >20 mg/dL and SOSM > 20 mOsm/kg. Continue until <20 or <10 mg/dL in the presence of endorgan damage.
Alkalinize to keep pH >7.35
IV thiamine 100 mg + pyridoxine 50 mg to increase metabolism of glyoxylate
Supportive, airway protection
NOTE: Isopropanol is metabolized by alcohol dehydrogenase to acetone, which is not an acid. Serum and urine “ketones” may be positive, but there is no metabolic acidosis or any increase in serum anion gap.
Dialysis indications
Level >50 mg/dL in association with severe metabolic acidosis or end-organ damage (use large dialyzer, blood flow > 300 mL/min). NOTE: 1. Fomepizole and ethanol are dialyzable. Dose adjustments are needed with dialysis. 2. Must monitor potassium, phosphorus, and magnesium and replace as needed.
Refractory and severe hemodynamic instability in association with levels >500 mg/dL and osmolal gap >100 mOsm/kg
Notes: Both methanol and ethylene glycol intoxication can give rise to a high serum anion gap metabolic acidosis, but isopropyl alcohol does not cause metabolic acidosis. Isopropyl alcohol is metabolized to acetone, which can result in positive serum and urine ketones, but since acetone is not an acid or anion, there should be no metabolic acidosis or elevated serum anion gap respectively.
Abbreviations: CNS, central nervous system; NO, nitric oxide; q, every; IV, intravenous.
Mechanisms of ketoacidosis in AKA:
Poor oral intake → ↓insulin and ↑glucagon, which lead to:
Increased lipolysis and free fatty acid (FFA) release from peripheral adipose tissues (lipase is normally inhibited by insulin)
Increased transport of FFA into mitochondria where FFA undergoes oxidation and ketone body formation
↓Insulin reduces ketone body utilization by insulin-sensitive tissues.
Elevated ratio of the reduced nicotinamide adenine dinucleotide (NADH) to NAD+ due to the metabolism of alcohol leads to:
Impaired conversion of lactate to pyruvate (increased lactate levels)
Impaired gluconeogenesis
A shift in hydroxybutyrate (β-OH) to acetoacetate (AcAc) equilibrium toward β-OH. In contrast to DKA, the predominant ketone body in AKA is β-OH. Routine clinical assays for ketonemia may only test for AcAc and acetone, but not for β-OH. Assays specific for the detection of β-OH will be necessary in suspected cases of AKA.
Volume depletion → ↓renal perfusion, reduced urinary excretion of ketoacids
Treatment:
Glucose + normal saline (dextrose [D5] increases insulin, stimulates oxidation of NADH to NAD+)
Thiamine to reduce risk of precipitating Wernicke encephalopathy or Korsakoff syndrome associated with glucose administration
Electrolytes monitoring and replacement (particularly those associated with refeeding syndrome, e.g., phosphorus, magnesium, potassium)
Occurs when hepatic glycogen stores are exhausted
Ketoacidosis is typically mild to moderate, with ketoanion levels of only 3 to 5 mmol/L. The ketone bodies produced in SKA, in turn, stimulate pancreatic islets to release sufficient insulin to control lipolysis, which renders SKA a self-limiting process. The AG reflects the ketoanion level, which also should not be very high.
The lack of insulin leads to increased mobilization of FFA from adipose tissue to the liver and hepatic lipid metabolism switch to ketogenesis.
Key points to remember in the management of DKA:
Excess hydration with normal saline and resultant high urine flow can lead to rapid urinary loss of ketone bodies, thereby converting a high SAG metabolic acidosis to a normal SAG metabolic acidosis (i.e., hyperchloremic metabolic acidosis). Ketone bodies are the source of rapid bicarbonate generation with insulin administration. The loss of ketone bodies may thus prolong the acidemic state. (The treatment of DKA in anuric dialysis patients typically only requires insulin administration and gentle fluid resuscitation to match poor oral intake and/or associated fluid loss, such as vomiting. The purpose of normal saline infusion in DKA is to primarily replace volume loss from osmotic diuresis and/or fluid loss from vomiting, not to “correct” the DKA. It is insulin administration that corrects DKA.)
Severe hypokalemia and hypophosphatemia may follow insulin administration and fluid resuscitation. In cases with life-threatening electrolyte deficiencies, repletion of these electrolytes must be done prior to insulin administration.
RTA may be suspected when there is a normal SAG metabolic acidosis, low urine ammonium level or positive urine anion gap (UAG), and urine osmolality gap (UOG) <100 mOsm/kg (Fig. 2.7).
A 24-hour urine ammonium level may be used in patients with normal AG metabolic acidosis to diagnose RTA.
Reference range for 24-hour urine ammonium is 15 to 56 mmol per Mayo Medical Laboratories.
Low urine ammonium (NH4+) and high urine pH suggest distal RTA (dRTA). In poor ammoniagenesis associated with hyperkalemia, urine pH may be low (see Distal RTA).
High urine ammonium (NH4+) and low urine pH suggest ongoing gastrointestinal (GI) losses. Patients with these findings are susceptible to uric acid and calcium oxalate stones.
If 24-hour urine NH4+ is not available, UAG may be used to estimate the NH4+ level. Simplified interpretation of UAG:
Assumptions:
The number of cations equals the number of anions in the urine.
Major cations in the urine include measured Na+, K+, and unmeasured NH4+ and other cations.
Major anions in the urine include measured Cl– and unmeasured HCO3– and other anions.
Thus,
Na+ + K+ + NH4+ + unmeasured cations = Cl– + HCO3– + unmeasured anions
Rearranging the equation,
and assuming negligible levels of unmeasured cations and anions:
Na+ + K+ – Cl– = HCO3– – NH4+
Interpretations:
In a patient with extrarenal metabolic acidosis (e.g., diarrhea), the kidneys will attempt to correct the underlying metabolic acidosis by increasing bicarbonate reabsorption (lower urine HCO3–) while increasing ammonium excretion (higher urine NH4+). This would result in a negative UAG.
Urine Na+ + K+ – Cl– = ↓HCO3– – ↑NH4+ <0
In contrast, in a patient with pRTA, bicarbonate wasting far exceeds maximal NH4+ excretion, hence positive UAG.
Urine Na+ + K+ – Cl– = ↑HCO3– – NH4+ >0
Urine Na+ + K+ – Cl– = HCO3– – ↓NH4+ >0
In toluene intoxication, there will be increased Na+ and K+ excretion along with the unmeasured hippurate/benzoate excretion. The quantity [Na+ + K+ – Cl–] will, therefore, be greater than 0 but may have nothing to do with RTA. Using the unsimplified equation above:
↑Na+ + ↑K+ – Cl– > 0
To understand the etiologies of pRTA, review normal bicarbonate reabsorption at the proximal tubules (Fig. 2.1). Any defect that interferes with normal bicarbonate reabsorption can lead to pRTA.
Isolated defects:
Autosomal recessive pRTA (SLCA4A4 gene) encoding NBC1: associated with mental retardation, ocular abnormalities (glaucoma, cataracts, band keratopathy)
Isolated defects are rare. Typically, pRTA is associated with other proximal tubular transport defects (Fanconi syndrome).
Fanconi syndrome: multiple proximal tubular transport defects leading to aminoaciduria, glucosuria, uricosuria, and phosphaturia
Genetic conditions associated with Fanconi syndrome: cystinosis, galactosemia, tyrosinemia, hereditary fructose intolerance, Lowe syndrome (oculocerebrorenal syndrome), Alport syndrome, Wilson disease, and mitochondrial cytopathies
Acquired conditions: multiple myeloma, heavy metals, amyloidosis, paroxysmal nocturnal hemoglobinuria, drugs (cisplatin, ifosfamide, aminoglycosides, imatinib, tenofovir, valproic acid), renal transplantation. Also see Chapter 6 Tubular, Interstitial, and Cystic Disorders.
Drugs that block CA II activity: acetazolamide, topiramate. and zonisamide (antiepileptic drugs). (Unlike pRTA from other causes where there is no increased stone risk, CA II inhibitor-induced pRTA may be associated with increased calcium phosphate and calcium oxalate stone risk. Reduced citrate excretion with these drugs is thought to be the stone-promoting factor.)
Bicarbonate replacement 5 to 15 mmol/kg/d (Note: Alkalinization can worsen hypokalemia)
Potassium replacement
Thiazide diuretics → induce volume contraction → enhance proximal reabsorption of bicarbonate. (Note: Thiazides can also worsen hypokalemia.)
Vitamin D and phosphate supplements for patients with rickets and hypophosphatemia
Metabolic acidosis may be severe, serum [HCO3–] may be much lower than 15 mmol/L.
Growth impairment
Polyuria
Hypercalciuria, nephrocalcinosis, nephrolithiasis: chronic metabolic acidosis increases bone resorption, thus hypercalciuria, and reduces urinary citrate excretion, thus hypocitraturia. The hypercalciuria, hypocitraturia, and high urine pH in most forms of dRTA facilitate calcium phosphate crystallization / stone formation. Also see Kidney Stones in Chapter 3.
Typically, patients with dRTA present with high urinary pH (e.g., >5.5). However, urinary pH could be less than 5.5 if ammoniagenesis is suboptimal. The latter may be seen in dRTA type 4 with concurrent hyperkalemia because hyperkalemia inhibits ammoniagenesis. NH3 is needed to buffer/facilitate H+ secretion: NH3 + H+ → NH4+. The lack of NH3 leaves more free H+ in tubular lumen, which lowers urine pH.
Of note, patients with dRTA type 4 do not have increased risk of nephrocalcinosis, which is likely due to their low urine pH.
To understand the etiologies of dRTA, review normal H+ secretion at the CCD (Fig. 2.2). Any defect that interferes with normal H+ secretion can lead to dRTA.
Normo-, hypokalemic dRTA:
Isolated defect/mutation of apical H+-ATPase: autosomal recessive (mutation of H+-ATPase β1-subunit may be associated with progressive sensorineural deafness)
Isolated defect/mutation of basolateral Cl–/HCO3– exchanger exchange (anion exchanger AE-1) in α-IC: autosomal dominant
Autoimmune diseases: Sjögren, rheumatoid arthritis, systemic lupus erythematosus, primary biliary cirrhosis, hypergammaglobulinemia.
Medications: lithium, ifosfamide (more commonly causes pRTA than dRTA), amphotericin, rarely bisphosphonates (zoledronate)
H+ back-leak: Amphotericin is a multiplanar lipophilic molecule that can insert itself into tubular membrane and act as an ionophore, allowing urinary H+ to leak back into tubular cells and circulation. Liposomal formulation of amphotericin essentially covers amphotericin with a lipophobic surface, thereby reducing the drug’s ability to insert itself into cell membrane (Fig. 2.8).
Aldosterone deficiency or aldosterone resistance, diabetic kidney disease, tubulointerstitial nephropathy, sickle cell nephropathy, obstructive uropathy
Medications: K-sparing diuretics (amiloride, triamterene), trimethoprim, pentamidine, calcineurin inhibitors (tacrolimus, cyclosporine), nonsteroidal anti-inflammatory drugs, inhibitors of the renin-angiotensin-aldosterone system (RAAS) (renin inhibitors, angiotensin-converting enzyme inhibitors [ACEIs], angiotensin-receptor blockers [ARBs], spironolactone, eplerenone), heparin
Factors affecting transmembrane voltage (e.g., obstructive uropathy, K-sparing diuretics)
Pseudohypoaldosteronism type 1 (PHA1) (ENaC mutation) and type 2 (i.e., Gordon syndrome, condition due to loss-of-function mutations of “with-no-lysine (K) kinase 1” (WNK1) or WNK4 resulting in NaCl cotransporter (NCC) hyperactivity and increased paracellular chloride reabsorption). See Hyperkalemia section.
Testing options:
NH4Cl (100 mg/kg lean body weight) oral load over 1 hour, or
Interpretation: Individuals with normal acid secretion can lower urine pH due to enhanced H+ secretion, but those with dRTA cannot.Stay updated, free articles. Join our Telegram channel
Full access? Get Clinical Tree
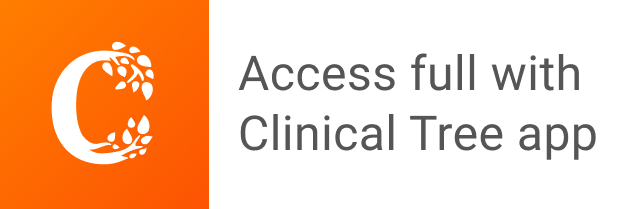