146 Bilal Chughtai,1 Dominique Thomas,1 & Alexis E. Te2 1 Department of Urology, Weill Cornell Medical College, New York Presbyterian Hospital, New York, NY, USA 2 Weill Medical College of Cornell University, New York, NY, USA Traditionally, the gold standard for treatment of benign prostatic hyperplasia (BPH) has been the electrocautery‐based transurethral resection of the prostate (TURP). TURP, however, is associated with several complications and side‐effects, including fluid absorption, electrolyte imbalance, intraoperative and postoperative bleeding, and inadequate resection. Laser therapy has several advantages over standard TURP, including technical simplicity and the absence or minimization of complications such as intraoperative fluid absorption and bleeding. Laser therapy has proven to result in shorter hospital stay and recovery for patients. Theoretically, less bleeding and irrigant absorption allows laser prostatectomy to treat larger glands with less physiologic stress, further suggesting a role for laser therapy in patients with a high burden of coexisting medical disease. An increasing number of practicing urologists are performing laser prostatectomies on patients with symptomatic BPH. Furthermore, the techniques and lasers used for laser prostatectomy are constantly evolving. To fully understand the mechanism of laser interaction with prostate tissue, some basic laser principles must first be understood. We will review the mechanisms of laser–tissue interaction and then provide a comprehensive review of the 532 nm laser prostatectomy. The word “laser” is derived from an acronym, standing for “light amplification by simulated emission of radiation” (LASER) [1]. An external energy source is used to excite electrons in a medium with an inherently large population of unstable electrons, causing the electrons to reach a higher energy state, called a metastable level. As the electrons return to ground state, they release an amount of energy equal to that initially absorbed, resulting in the spontaneous emission of photons [1–3]. Unlike normal white light, which is made up of the entire visible electromagnetic spectrum, laser energy is monochromatic (i.e. all of its photons originate from the same energy level transition with both spatial and temporal coherence) [1–3]. In addition, laser rays are parallel to each other and hence do not converge or diverge unless reflected or focused by a lens or mirror. However, when transmitted through flexible fibers by internal reflection, the laser beam will become divergent as it exits the fiber. Laser energy affects tissue by inducing coagulation, vaporization, or even a mechanical tearing of tissue [4]. Power directly influences the temperature level reached and the speed with which a surgical effect is achieved. In general, a temperature of greater than 50°C is required to produce a coagulative effect, while a temperature of greater than 100°C is required to produce a vaporization effect. Medical laser sources can possess a number of power outputs ranging from 20 to 180 W. Power density, defined as power per unit area (P/A), represents the most important parameter that expresses the strength of the laser at a fixed location. Fibers with narrowly divergent beams will have a much higher power density than fibers with widely divergent beams due to the difference in the size of target areas. As a corollary, this also implies that maximum power density arises when the tip of a laser fiber is almost in contact with tissue (i.e. at the distance where divergence is minimized) [1–3, 5, 6]. Four major interactions occur when laser energy is applied to human tissue: reflection, scattering, penetration, and absorption. Up to 50% of the incident energy of a laser beam is reflected away. After the initial reflection, the remaining energy is then transmitted through the tissue for a specific distance, depending upon the type of laser used as well as the particular type of tissue being treated. After absorption, the laser energy is then converted to thermal energy, or heat. As laser energy strikes the irregularities of a tissue surface, diffuse reflection, also known as scattering, occurs. Scattering may occur in either forward or backward directions. The amount of scatter that occurs depends on tissue characteristics such as water content, cell constituents, and pigments. Scattering also depends on the resonant absorption and re‐emission of radiation by the atoms of the receiving tissue, as well as the diffraction or radiation of laser energy in directions away from the line of propagation. As a result of scattering, a laser beam does not merely continue to penetrate in a straight line but dissipates its energy within the surrounding area, beginning when it hits the first tissue interface and continuing to create a zone of heat along the path of the beam. Alternatively, laser energy may penetrate tissue. The depth of penetration depends on its wavelength and the composition of the receiving tissue. Penetration is often described by “extinction length,” the depth of penetration of an incident beam beyond which only 10% of the initial beam energy is left (i.e. 90% absorption). Thus, after one extinction length, 10% of the beam will penetrate further, whereas after two extinction lengths, 1% of the beam will penetrate further, and so on. Finally, laser energy may be absorbed by tissue. Absorption of laser energy is often described by “absorption length,” the depth of tissue through which 63% of the incident beam energy is absorbed. It is important to note that the different components of living tissue absorb different laser energy wavelengths in variable fashions. Water, constituting 75–85% of soft tissue, absorbs 532 nm laser energy very poorly, resulting in deep penetration of the laser beam into tissue. Pigments such as hemoglobin, bilirubin, and melanin absorb 532 nm energy very efficiently. Finally, carbon, an abundant constituent of all living tissue, is a final breakdown product of pyrolysis and represents a strong absorber of all wavelengths of laser energy. The original 532 nm laser was a potassium titanyl phosphate (KTP)‐based laser system that contained a KTP crystal through which a 532 nm wavelength was generated. This had a different interaction with prostate tissue from its parent, the neodymium:yttrium–aluminum–garnet (Nd:YAG) [7]. The 532 nm wavelength is selectively absorbed by hemoglobin, which acts as an intracellular chromophore. The 532 nm laser energy can be fully transmitted through aqueous irrigants into the cell, where it is absorbed by hemoglobin, which is then rapidly heated, leading to vaporization of prostate tissue. The short optical penetration associated with this wavelength confines its high‐power laser energy to a superficial layer of prostate tissue that is vaporized rapidly and hemostatically, with only a 1–2 mm rim of coagulation. The thin coagulation zone arises as a result of the quasi‐continuous emission characteristics of the 532 nm laser. Typically, continual irradiation of a single point causes heat to diffuse into deeper tissue layers, causing coagulation wherever there is enough convection thermal energy for protein denaturation but insufficient energy for vaporization. These selective characteristics led to the use of the 532 nm laser in prostatectomy as “photoselective vaporization of the prostate” (PVP). Some of the first studies designed to test the efficacy of higher power 60 W KTP lasers first began their experimentation with both in vivo and cadaveric canine studies [8]. These initial canine studies were capable of providing evidence to support the 60 W laser’s ability in not only vaporizing the tissue, but minimizing any simultaneous coagulation effects. Malek et al. described the first human trials in 10 patients using the 60 W KTP laser [9]. Mean operating time for the procedure was approximately 29 minutes. The results demonstrated no postoperative incidences of urinary retention and no transurethral resection syndrome. This study showed all patients were catheter‐free within 24 hours of the procedure. After 24 hours, maximum renal flow rate (Qmax) had significantly improved by 142% (8 ± 1.3 ml/s vs. 19.4 ± 8.4 ml/s, P = 0.003266). These initial results suggested promise at three months follow‐up. In 2000, a larger study of 55 patients followed patients using the 60 W KTP laser [10]. Mean operating time was 44 minutes. At 2 years follow‐up patients showed statistically significant improvements in postoperative American Urological Association Symptom Index (AUA‐SI) scores (mean 14, 82% improvement), Qmax (mean 29.1 ml/s, 278% improvement), and PVR (27 ml, 75% improvement). After the procedure, all patients were catheter‐free within 24 hours. Furthermore, no patients required recatheterization, nor did anyone experience transurethral resection syndrome. Although many patients used antiplatelet agents (at least half), hematuria was negligible. Overall, the results showed that PVP with 60 W KTP was as effective as TURP. The use of 60 W KTP was less than ideal due to its low power, which limited the size of the prostate that could be resected. In order to achieve the desired tissue ablation, the 80 W KTP laser system was introduced. It is interesting to note that to preserve a thin coagulation zone while maintaining high vaporization efficiency, laser pulsing was incorporated into the 80 W higher power KTP laser system. A high‐frequency modulation of laser light generates a continuous stream of short micropulses with a duration of 4.5 ms and a peak power of 280 W (i.e. 3.5 times the average laser power of the regular 80 W laser). The short duration of the micropulses does not allow time for heat to diffuse from the superficial layer, thus confining energy to a small volume of tissue, a situation referred to as thermal confinement. As each micropulse generates a very fast temperature increase inside tissue, the tissue water is not only rapidly vaporized but the surrounding tissue matrix is torn apart, allowing for efficient removal of prostate tissue. Continuous bladder irrigation is thus required to cool the tissue as well as to provide a clear aqueous medium for laser light to be transmitted to target tissue without energy loss. Initial experiments utilizing the 80 W KTP laser began with with ex vivo animal models [11]. Twenty perfused porcine kidneys were used to model human prostate tissue. The high‐power resection was compared to traditional TURP‐like resection and it was found that 80 W KTP showed a statistically significant decrease in hemorrhage (P < 0.0001). This demonstrated that bloodless ablation of tissue was possible. Hai and Malek investigated the first human trials using 80 W KTP laser prostatectomy [12]. A total of 10 patients were followed for 1 year postoperatively. Mean operating time was 19.8 minutes. Patients experienced statistically significant improvements in AUA‐SI score (23.2–2.6), quality‐of‐life (QOL) scores (4.3–0.5), Qmax (10.3–30.7 ml/s), and postvoid residual volume (PVR) (137.6–3 ml). Furthermore, there was a 27% reduction in prostate volume. No patients in the study experienced postoperative urinary retention, infection, incontinence, or erectile dysfunction; none subsequently developed bladder neck contractures or urethral stricture. Interestingly, two patients did not require catheterization following the procedure. One patient actively taking anticoagulants did experience mild transient postoperative hematuria, which required recatheterization for 24 hours. De Nunzio et al. showed in 150 patients that 80 W KTP laser was effective in decreasing storage and voiding symptoms of 81.8% and 90.9%, respectively, at 12 months [13]. Consistent with other published series, these studies further support the procedure’s safety, efficacy, and durable improvements on International Prostate Symptom Score (IPSS) and QOL [14]. The 80 W KTP/532 nm laser was also tested on large prostates by Sandhu et al. [15]. Sixty‐four men with BPH and prostates with volumes of at least 60 ml (mean 101 ml) underwent vaporization with the 80 W KTP laser. The mean operative time was 123 minutes. The IPSS decreased from 18.4 to 6.7 at 12 months; Qmax increased from 7.9 to 18.9 ml/s, while PVR decreased from 189 to 109 ml. No transfusions were required nor was there evidence of postoperative hyponatremia. All 62 patients were discharged within 23 hours. Pfitzenmaier et al. conducted a comparative study between vaporization of prostates greater than or equal to 80 ml (39 of 173 patients) and those smaller than 80 ml [16]. PVP was discovered to be safe and effective in prostates greater than or equal to 80 ml [16]. One caveat was that reoperation was higher in the larger prostate cohort. Another study by Rajbabu et al. evaluated 54 patients with prostates greater than 100 ml who underwent 80 W KTP laser prostatectomy [14]. The mean operating time was 81.6 minutes. The mean catheterization for patients was 23.0 hours. Mean urinary flow rate improved from 8.0 to 19.3 ml/s from three months to 24 months postoperatively [14]. PVP, IPSS, QOL, prostate specific antigen (PSA), and prostate volume all showed statistically significant improvements. No complications were reported. In one study, 76 patients with a prostate size of greater than 70 ml were randomized to TURP or 80 W laser prostatectomy [17]. Procedure time was shorter for the TURP group. Hospitalization stay and catheterization time were significantly shorter in the laser group. TURP favored prostate volume reduction as well as significant improvements in IPSS, PVR, and Qmax. In addition, reoperation rate was higher in the laser group. Ruszat et al. randomized 396 patients to either 80 W laser prostatectomy or TURP [18]. Interim 24 months follow‐up data found the rates of intraoperative bleeding (3% vs. 11%), blood transfusion (0% vs. 5.5%), capsule perforations (0.4% vs. 6.3%), and early postoperative clot retention (0.4% vs. 3.9%) were significantly lower in the laser group. There was no significant difference in IPSS and PVR. After 12 months, size reduction was greater in the TURP group (66% vs. 44%) and the rate of repeat procedure was greater in the PVP group (6.9% vs. 3.9%, not significant). Bouchier‐Hayes et al. reported data on 120 patients randomized to undergo TURP or 80 W laser PVP [19]. At 12 months equivalent improvements in IPSS and flow rates were demonstrated. Length of hospitalization, length of catheterization, and adverse events were lower in the laser group. In a nonrandomized study, Bachmann et al. studied 101 patients who underwent either TURP or laser prostatectomy. Perioperative morbidity and symptom improvement was equivalent in the groups at six months [20]. An Australian study had similar results when comparing patients randomized to either 80 W laser prostatectomy or TURP [21]. Both groups showed a significant increase in mean urinary flow rate, improvement in IPSS scores, and no difference in sexual function at 1‐year follow‐up. Guo et al. conducted a study evaluating 5‐year follow‐up after the use of 80 W KPT GreenLight laser vaporization of the prostate in comparison to TURP [22]. The purpose of this study was to compare long‐term results of both procedures in comparison to safety, efficacy and long‐term durability. This was a two‐center nonrandomized prospective study. One hundred and twenty patients underwent PVP and 80 underwent TURP. At 60 months follow‐up 30 patients from the PVP arm and 31 patients from the TURP arm were available for analysis [22]. There were no statistically significant differences in IPSS, QOL, Qmax, and PVR between the two groups [22]. Those receiving 80 W KTP had a higher reoperation rate due to urethral stricture, bladder neck contracture, and persisting and recurrent adenoma [22]. There have been a number of studies evaluating the long‐term outcomes of 80 W KTP laser prostatectomy. Te et al. investigated the use of the 80 W KTP laser in laser prostatectomy in the first large multicenter study. A total of 145 patients were included in the study with long‐term follow‐up [23]. Overall, there were significant and durable improvements in AUASI scores, QOL scores, Qmax, and PVR 12 months postoperatively. Mean AUA‐SI scores declined from 24 to 1.8 at 12 months; mean QOL scores improved from 4.3 to 0.4, Qmax from 7.7 to 22.8 ml/s, and PVR from 114.2 to 7.2 ml. Mean prostate volume, as determined by ultrasound, decreased from 54.6 to 34.4 ml. The mean operation time was 36 minutes with no patient requiring a blood transfusion. More than 30% of patients were sent home without a catheter; those with postoperative catheters had them removed in a mean of 14 hours. Approximately 8% of patients experienced mild‐to‐moderate dysuria that lasted more than 10 days, 8% had transient hematuria, and 3% had postoperative retention. Of the 56 men who were potent prior to the procedure, 27% experienced retrograde ejaculation but none of them experienced impotence. Ruszat et al. published the largest series of 80 W KTP laser prostatectomies. At a single center, 500 patients underwent PVP, including 45% taking oral. Three years postoperatively, 26.2% of patients were included in the analysis. Mean AUA‐SI, PVR, and QOL were significantly improved compared to results at baseline. At 60 months, the retreatment rate was 6.8% and reoperation rate was 14.8%. Urethral and bladder neck strictures were observed in 4.4% and 3.6% of patients, respectively, comparable to the rates in TURP [24]. Te et al. reported a multicenter long‐term follow‐up in 139 patients who underwent 80 W KTP laser prostatectomy. At 3 years, 33.8% of patients were included in the follow‐up and improvements in symptom relief and urinary flow rate were durable [25]. The overall retreatment rate for this cohort was 4.3%. The safety of 80 W KTP laser prostatectomy has been studied in patients at high cardiopulmonary risk, and demonstrated excellent results due to a robust hemostatic profile and perioperative hemodynamic stability of the procedure. Reich et al. performed 80 W laser prostatectomy on 66 patients with an American Society of Anesthesiology Score of 3 or greater [26]. Of these patients, 29 were being treated with ongoing oral anticoagulant or had a severe bleeding disorder. Throughout the study, no patients presented with major complications during or following the procedure and no patients required blood transfusions. Despite this, within 12 months, two patients required reoperation due to recurrent urinary retention. Mean improvements in IPSS (from 20.2 to 6.5) and peak flow (from 6.7 to 21.6 ml/s) were durable at 12 months. The final safety aspect of the 80 W KTP laser to be studied in detail was its use in anticoagulated patients at high risk for clinically significant bleeding. Twenty‐four anticoagulated patients with BPH were treated with laser prostatectomy using the 80 W KTP laser [27]. Eight were on warfarin, 2 on clopidogrel, and 14 on aspirin. Eight (33%) of these patients had had a previous myocardial infarction; 7 (29%) cereberovascular disease, and 7 (29%) peripheral vascular disease. No patients developed clinically significant hematuria postoperatively and none developed clot retention. No patients required blood transfusions, nor were there any thromboembolic events. Follow‐up revealed a decrease in IPSS from 18.7 to 9.5, as well as an increase in Qmax from 9.0 to 20.1 ml/s at 12 months. PVR decreased from 134 to 69 ml at one month but this was not statistically significant beyond that time point. All patients underwent PVP safely without any adverse thromboembolic or bleeding events. However, more energy and time were required for lasing per gland size in these patients [28]. The 120 W is similar to the 80 W KTP laser system, but it evolved to a higher power system (HPS) capable of 80–120 W. The laser emits the same 532 nm wavelength, with the same hemostatic properties as the 80 W KTP laser [29], but uses a different crystal. The 532 nm 80 W KTP laser is created by passing a 1064 nm Nd:YAG laser beam through a KTP crystal. In contrast, the 120 W HPS 532 nm wavelength is created by passing a Nd:YAG laser beam through a lithium triborate (LBO) crystal. The 532 nm LBO‐based system also has a beam that is better collimated than the KTP beam. The use of the 120 W 532 nm laser was first evaluated using animal models. Lee et al. first investigated the use of the 120 W laser in five male beagles [30]. PVP was performed in antegrade fashion through a suprapubic cystotomy at 40, 80, and 120 W settings for three distinct firing periods (5, 10, and 20 seconds) at unique locations in the prostate. The 120 W HPS consistently vaporized more tissue per unit time, while the depth of coagulation (1.2–2.5 mm) was decreased compared to the lower powered systems. Kang et al. compared the use of the 120 W HPS laser to the 80 W HPS laser in 96 specimens of bovine prostate tissue [31]. The 120 W HPS laser vaporized bovine prostate tissue more efficiently than the 80 W KTP laser and coagulation was equivalent. Lee et al. advised caution with the higher power setting, especially at 120 W due to the potential higher risk of capsular perforation and bladder wall perforation [30]. In addition, higher power provides more efficient vaporization with less hemostasis, and as a result, utilization of the lower power coagulation setting is important in achieving hemostasis. Heinrich et al. used blood‐perfused porcine kidneys to determine the ablation capacity, hemostatic properties, and coagulation depth of a 120 W 532 nm laser compared to an 80 W laser [32]. The 120 W LBO laser offered a significantly higher tissue ablation capacity compared with the conventional 80 W KTP laser. The increased efficacy of the 120 W laser device was accompanied by a higher bleeding rate and a slightly deeper coagulation zone. Woo et al. published one of the first studies evaluating the efficacy of 120 W 532 nm laser prostatectomy in humans [33]. In a multicenter prospective study, 305 patients with BPH underwent laser prostatectomy using the 120 W HPS laser. Study results showed an increase in Qmax as well as decreases in PVR, IPSS scores, and prostate volume from baseline to follow‐up. All of these parameters were significant (P < 0.001) in all patients. A subgroup analysis was done in three groups of patients: those in retention (n = 63), on anticoagulation (n = 67), and with prostates ≥80 ml (n = 52). Complications were comparatively low in all groups. In another study by Al‐Ansari et al. 120 patients with BPH were randomized to either TURP or 120 W 532 nm laser [34]. Baseline characteristics for both groups were comparable. After 36 months of follow‐up, a total of 55 patients in TURP and 54 patients in 532 nm laser groups completed the study. The mean operative time was significantly shorter for TURP. Compared to preoperative values, there was significant reduction in hemoglobin and serum sodium levels at the end of TURP only. In the PVP, no major intraoperative complications were recorded and none of the patients required blood transfusion. Of those patients in the TURP group, “12 (20%) required transfusion, 3 (5%) developed transurethral resection syndrome, and capsule perforation was observed in 10 patients” [34]. Significant improvement was observed in Qmax, IPSS, and PVP compared with preoperative values. The degree of improvement was comparable in both groups at all time points of follow‐up. Storage bladder symptoms were significantly higher in PVP. Reoperation was required in one TURP patient and six PVP patients (P < 0.05). Lukacs et al. conducted a similar study using GreenLight HPS 120 W and TURP. A total of 70 men were randomized to receive 120 W and 69 were randomized to receive TURP [35]. IPSS scores could not be demonstrated to be noninferior. The mean length of hospital stay was significantly shorter in those in the PVP group 1 (interquartile range (IQR) 1–2) versus 2.5 (IQR 2–3.5), respectively (P < 0.0001) [35]. Other uroflowmetry clinical values were comparable across both groups [35]. Zang et al. conducted 3‐year follow‐up after 78 patients underwent PVP using a 120 W system [36]. The patient’s urinary characteristics and QOL scores drastically improved following the procedure. “At 36 months, the average percentage of improvements compared with baseline was as follows: IPSS, 60.2%; QOL, 80.9%; Qmax, 138.7%, and PVR, 82.6%” [36]
532 nm High‐power Transurethral Laser Prostatectomy
Introduction
Laser physics
Laser–tissue interaction
Reflection
Scattering
Penetration
Absorption
History of the 532 nm laser
The 60 W laser systems
The 80 W laser systems
The 120 W laser systems
Stay updated, free articles. Join our Telegram channel
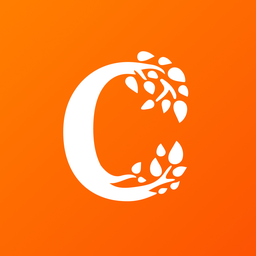
Full access? Get Clinical Tree
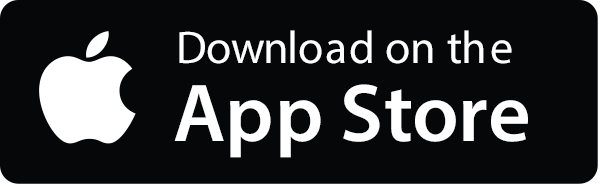
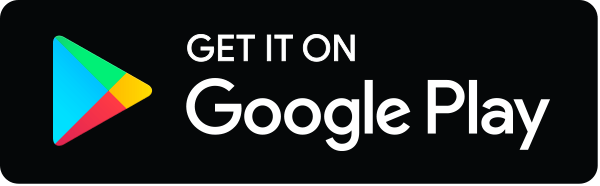